28.1 Carbohydrates
Learning Objectives
By the end of this section, you will be able to:
- Recognize carbohydrates and classify them as mono-, di-, or polysaccharides
- Classify monosaccharides as aldoses or ketoses and as trioses, tetroses, pentoses, or hexoses
- Distinguish between a D sugar and an L sugar
- Identify the structures of D-glucose, D-galactose, and D-fructose and describe how they differ from each other
- Define what is meant by anomers and describe how they are formed
- Explain what is meant by mutarotation
- Identify the chemical properties of monosaccharides
- Identify the structures of sucrose, lactose, and maltose
- Identify the monosaccharides that are needed to form sucrose, lactose, and maltose
- Compare and contrast the structures and uses of starch, glycogen, and cellulose.
Carbohydrates: an Important Source of Energy
Carbohydrates, often referred to as the body’s primary source of energy, have played a pivotal role in the history of nutrition and human evolution. Throughout history, carbohydrates from sources like grains, fruits, and vegetables have provided sustenance and fueled societies, enabling the growth of civilizations. In the modern era, the study of carbohydrates has revealed their crucial role in maintaining health, regulating blood sugar levels, and supporting bodily functions. From the complex polysaccharides found in whole grains to the simple sugars in fruits, carbohydrates offer a wide spectrum of nutrients essential for human well-being. Understanding the significance of carbohydrates not only contributes to a balanced diet but also promotes a healthier, more energy-efficient lifestyle, making them an indispensable component of nutrition science and public health.
All carbohydrates consist of carbon, hydrogen, and oxygen atoms and are polyhydroxy aldehydes or ketones (as seen in Figure 28.1a.) or are compounds that can be broken down to form such compounds. Examples of carbohydrates include starch, fiber, the sweet-tasting compounds called sugars, and structural materials such as cellulose. The term carbohydrate had its origin in a misinterpretation of the molecular formulas of many of these substances. For example, because its formula is C6H12O6, glucose was once thought to be a “carbon hydrate” with the structure C6·6H2O.
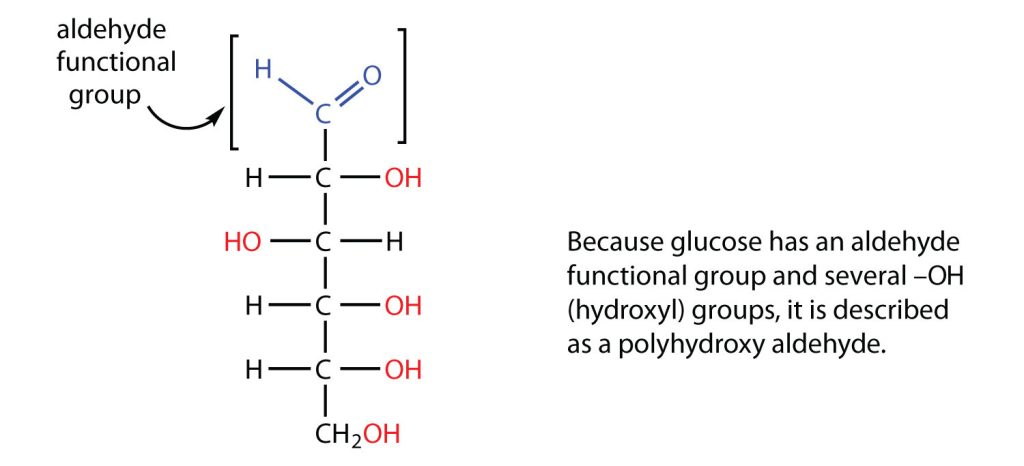
Green plants are capable of synthesizing glucose (C6H12O6) from carbon dioxide (CO2) and water (H2O) by using solar energy in the process known as photosynthesis:
6 CO2 + 6 H2O + 686 kcal → C6H12O6 + 6 O2
(The 686 kcal come from solar energy.) Plants can use the glucose for energy or convert it to larger carbohydrates, such as starch or cellulose. Starch provides energy for later use, perhaps as nourishment for a plant’s seeds, while cellulose is the structural material of plants. We can gather and eat the parts of a plant that store energy—seeds, roots, tubers, and fruits—and use some of that energy ourselves. Carbohydrates are also needed for the synthesis of nucleic acids and many proteins and lipids.
Animals, including humans, cannot synthesize carbohydrates from carbon dioxide and water and are therefore dependent on the plant kingdom to provide these vital compounds. We use carbohydrates not only for food (about 60%–65% by mass of the average diet) but also for clothing (cotton, linen, rayon), shelter (wood), fuel (wood), and paper (wood).
Spotlight on Everyday Chemistry: Diabetes Mellitus
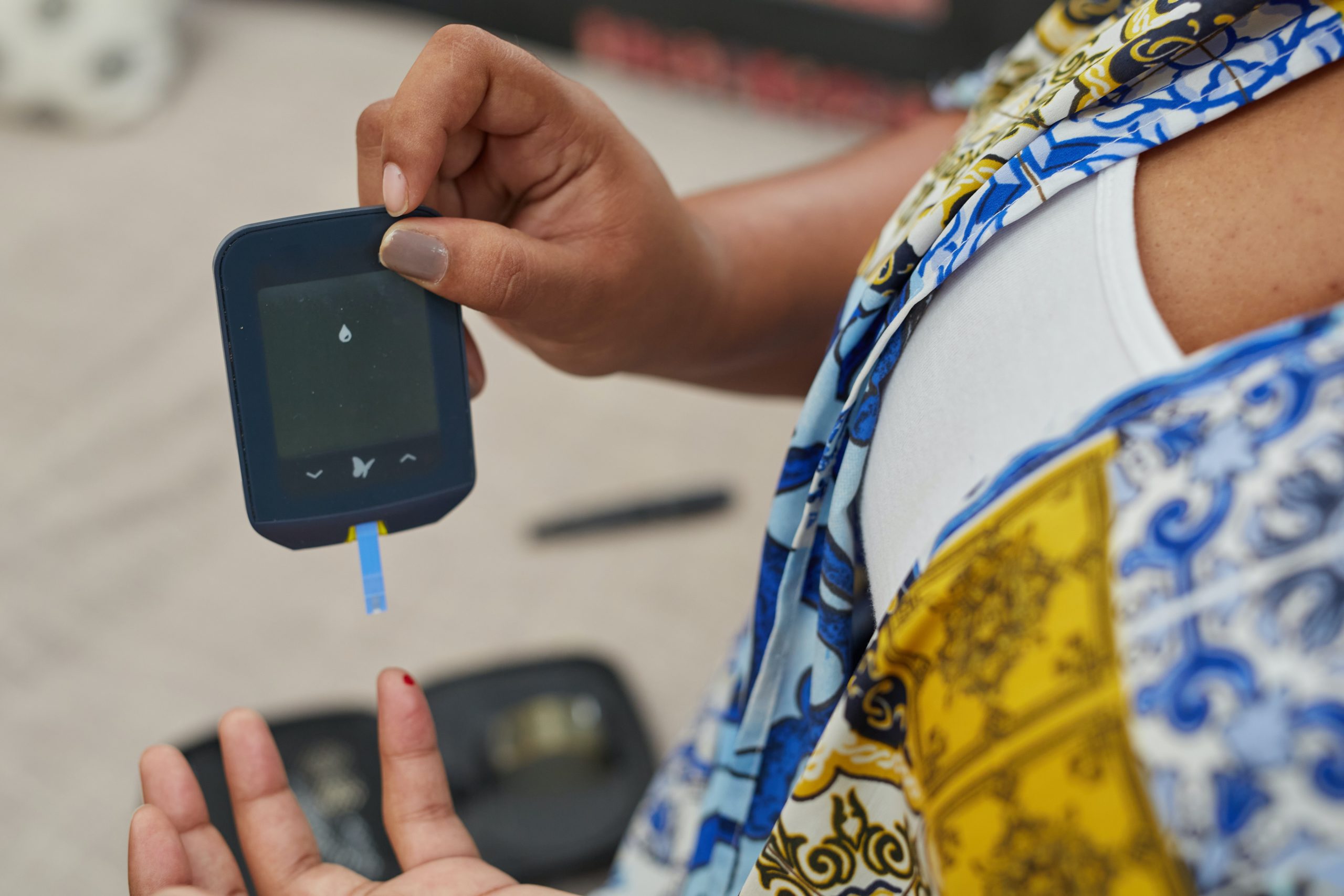
In the United States, 17.9 million people have been diagnosed with diabetes, and experts estimate that at least another 5.7 million people have the disease but have not been diagnosed. In 2006, diabetes was the seventh leading cause of death, listed on 72,507 death certificates. Moreover, it was a contributing factor in over 200,000 deaths in which the cause was listed as something else, such as heart or kidney disease.
People with diabetes are impaired in their ability to metabolize glucose, a sugar needed by the body for energy; as a result, excessive quantities of glucose accumulate in the blood and the urine. The characteristic symptoms of diabetes are weight loss, constant hunger, extreme thirst, and frequent urination (the kidneys excrete large amounts of water in an attempt to remove the excess sugar from the blood).
An important diagnostic test for diabetes is the oral glucose tolerance test, which measures the level of glucose in blood plasma. A first measurement is made after a fast of at least 8 h, followed by another measurement 2 h after the person drinks a flavoured solution of 75 g of glucose dissolved in water. At the second measurement, the glucose plasma level should be no higher than 139 mg/dL. Individuals with a value between 140 and 199 mg/dL are diagnosed with prediabetes, while those with a value of 200 mg/dL or above are diagnosed with diabetes. Following a diagnosis of diabetes, a person will need to monitor his or her blood glucose levels daily (or more often) using a glucose meter.
The simplest carbohydrates—those that cannot be hydrolyzed to produce even smaller carbohydrates—are called monosaccharides. Two or more monosaccharides can link together to form chains that contain from two to several hundred or thousand monosaccharide units. Prefixes are used to indicate the number of such units in the chains. Disaccharide molecules have two monosaccharide units, trisaccharide molecules have three units, and so on. Chains with many monosaccharide units joined together are called polysaccharides. All these so-called higher saccharides can be hydrolyzed back to their constituent monosaccharides. Compounds that cannot be hydrolyzed will not react with water to form two or more smaller compounds.
Classes of Monosaccharides
The naturally occurring monosaccharides contain three to seven carbon atoms per molecule. Monosaccharides of specific sizes may be indicated by names composed of a stem denoting the number of carbon atoms and the suffix –ose. For example, the terms triose, tetrose, pentose, and hexose signify monosaccharides with, respectively, three, four, five, and six carbon atoms. Monosaccharides are also classified as aldoses or ketoses. Those monosaccharides that contain an aldehyde functional group are called aldoses; those containing a ketone functional group on the second carbon atom are ketoses. Combining these classification systems gives general names that indicate both the type of carbonyl group and the number of carbon atoms in a molecule. Thus, monosaccharides are described as aldotetroses, aldopentoses, ketopentoses, ketoheptoses, and so forth. Glucose and fructose are specific examples of an aldohexose and a ketohexose, respectively, as shown in Figure 28.1c.
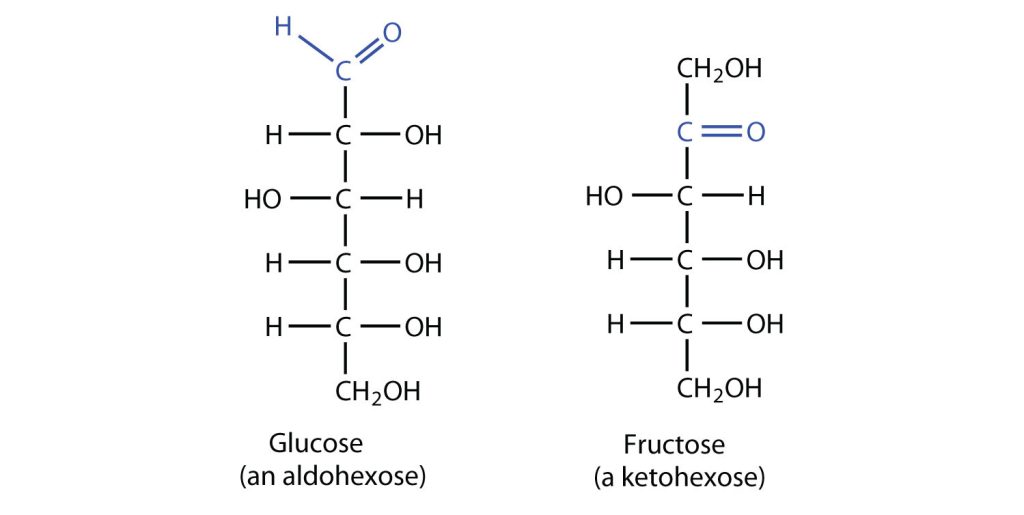
Draw an example of each type of compound.
- a ketopentose
- an aldotetrose
Solution
- The structure must have five carbon atoms with the second carbon atom being a carbonyl group and the other four carbon atoms each having an OH group attached. Several structures are possible, but one example is shown:
- The structure must have four carbon atoms with the first carbon atom part of the aldehyde functional group. The other three carbon atoms each have an OH group attached. Several structures are possible, but one example is shown:
Example source: Introduction to Chemistry: GOB (V. 1.0)., CC BY-NC-SA 3.0.
Exercise 28.1a
Examining Figure 28.1c, identify the main functional groups in each molecule. List some of the physical properties of each functional group.
Check Your Answer: [1]
Source: Exercise 28.1a by Samantha Sullivan Sauer is licensed under CC BY-NC 4.0.
The simplest sugars are the trioses. The possible trioses are shown in part (a) of Figure 28.1d: glyceraldehyde is an aldotriose, while dihydroxyacetone is a ketotriose. Notice that two structures are shown for glyceraldehyde. These structures are stereoisomers, and hence are isomers having the same structural formula but differing in the arrangement of atoms or groups of atoms in three-dimensional space. If you make models of the two stereoisomers of glyceraldehyde, you will find that you cannot place one model on top of the other and have each functional group point in the same direction. However, if you place one of the models in front of a mirror, the image in the mirror will be identical to the second stereoisomer in part (b) of Figure 28.1d. Molecules that are nonsuperimposable (nonidentical) mirror images of each other are a type of stereoisomer called enantiomers (Greek enantios, meaning “opposite”). It is important to note that these are another type of stereoisomer than the cis-trans (geometric) isomers previously discussed.
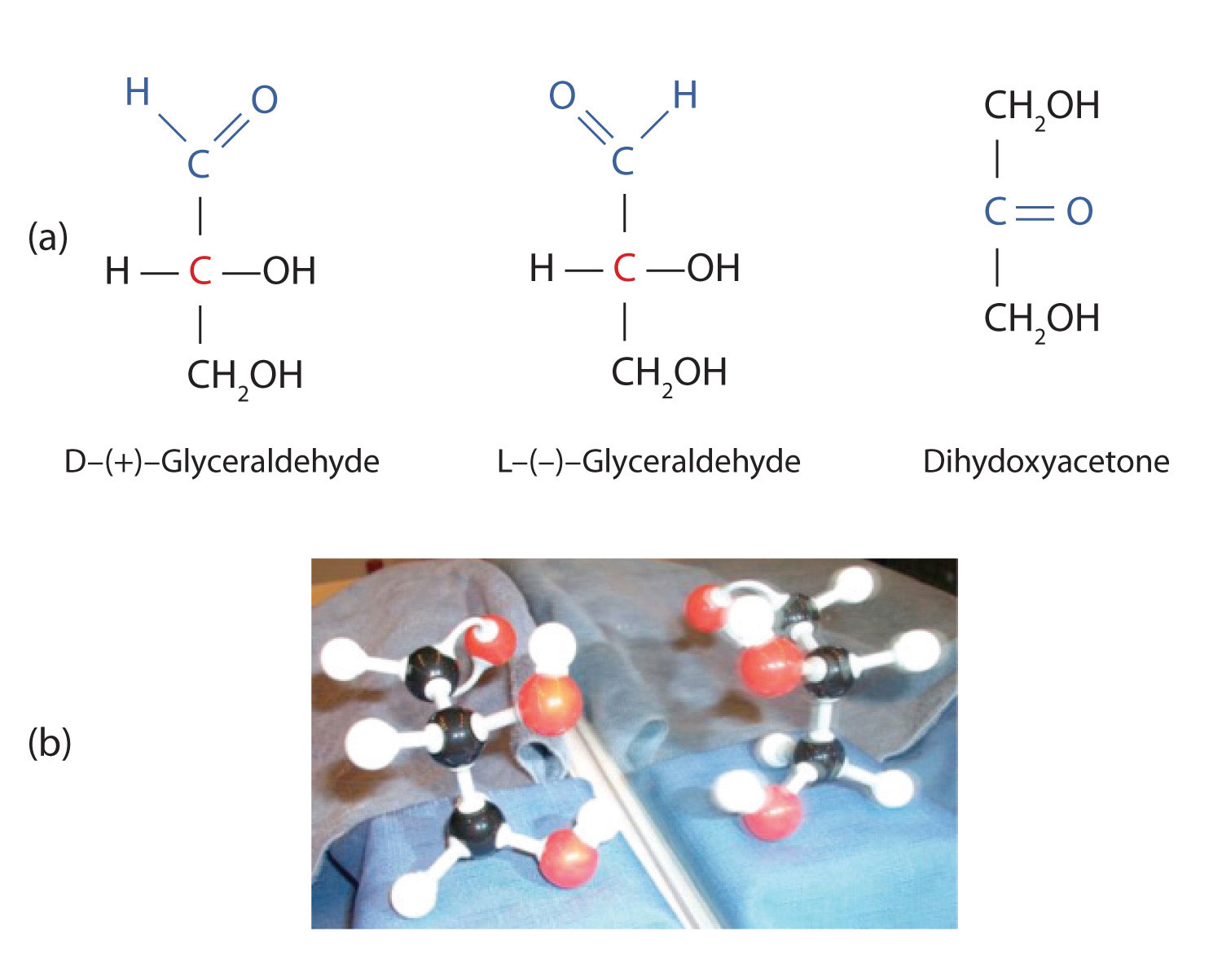
A key characteristic of enantiomers is that they have a carbon atom to which four different groups are attached. Note, for example, the four different groups attached to the central carbon atom of glyceraldehyde (part (a) of Figure 28.1d.). A carbon atom that has four different groups attached is a chiral carbon. If a molecule contains one or more chiral carbons, it is likely to exist as two or more stereoisomers. Dihydroxyacetone does not contain a chiral carbon and thus does not exist as a pair of stereoisomers. Glyceraldehyde, however, has a chiral carbon and exists as a pair of enantiomers. Except for the direction in which each enantiomer rotates plane-polarized light, these two molecules have identical physical properties. One enantiomer has a specific rotation of +8.7°, while the other has a specific rotation of −8.7°.
H. Emil Fischer, a German chemist, developed the convention commonly used for writing two-dimensional representations of the monosaccharides, such as those in part (a) of Figure 28.1d. In these structural formulas, the aldehyde group is written at the top, and the hydrogen atoms and OH groups that are attached to each chiral carbon are written to the right or left. (If the monosaccharide is a ketose, the ketone functional group is the second carbon atom.) Vertical lines represent bonds pointing away from you, while horizontal lines represent bonds coming toward you. The formulas of chiral molecules represented in this manner are referred to as Fischer projections.
The two enantiomers of glyceraldehyde are especially important because monosaccharides with more than three carbon atoms can be considered as being derived from them. Thus, D- and L-glyceraldehyde provide reference points for designating and drawing all other monosaccharides. Sugars whose Fischer projections terminate in the same configuration as D-glyceraldehyde are designated as D sugars; those derived from L-glyceraldehyde are designated as L sugars. By convention, the penultimate (next-to-last) carbon atom has been chosen as the carbon atom that determines if a sugar is D or L. It is the chiral carbon farthest from the aldehyde or ketone functional group.
A beam of ordinary light can be pictured as a bundle of waves; some move up and down, some sideways, and others at all other conceivable angles. When a beam of light has been polarized, however, the waves in the bundle all vibrate in a single plane. Light altered in this way is called plane-polarized light. Much of what chemists know about stereoisomers comes from studying the effects they have on plane-polarized light. In Figure 28.1e., the light on the left is not polarized, while that on the right is polarized.
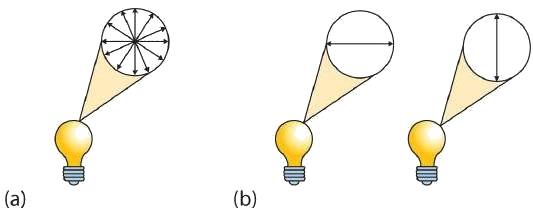
Sunlight, in general, is not polarized; light from an ordinary light bulb or an ordinary flashlight is not polarized. One way to polarize ordinary light is to pass it through Polaroid sheets, special plastic sheets containing carefully oriented organic compounds that permit only light vibrating in a single plane to pass through. To the eye, polarized light doesn’t “look” any different from nonpolarized light. We can detect polarized light, however, by using a second sheet of polarizing material, as shown in Figure 28.1f.
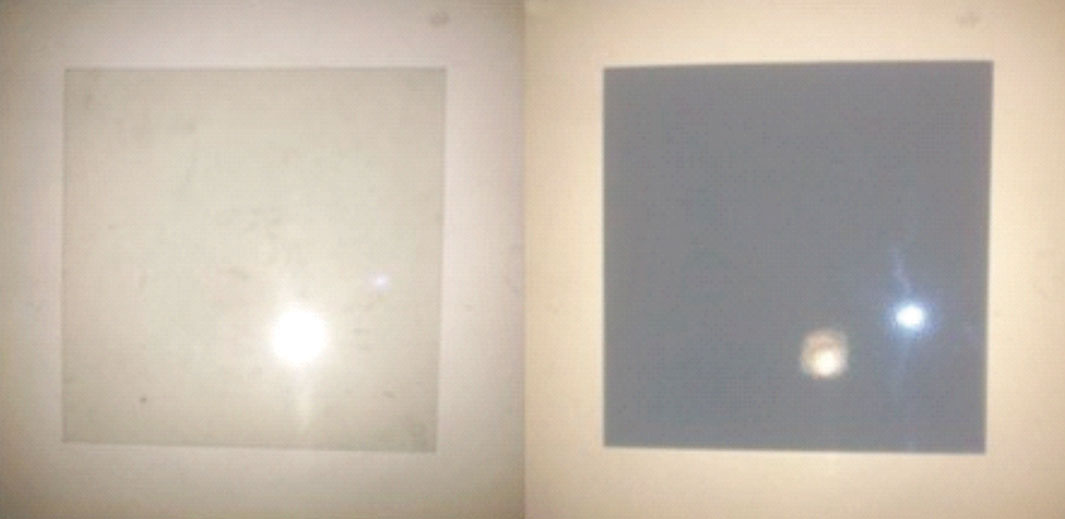
Certain substances act on polarized light by rotating the plane of vibration. Such substances are said to be optically active. The extent of optical activity is measured by a polarimeter, an instrument that contains two polarizing lenses separated by a sample tube, as shown in Figure 28.1g. With the sample tube empty, maximum light reaches the observer’s eye when the two lenses are aligned so that both pass light vibrating in the same plane. When an optically active substance is placed in the sample tube, that substance rotates the plane of polarization of the light passing through it, so that the polarized light emerging from the sample tube is vibrating in a different direction than when it entered the tube. To see the maximum amount of light when the sample is in place, the observer must rotate one lens to accommodate the change in the plane of polarization.
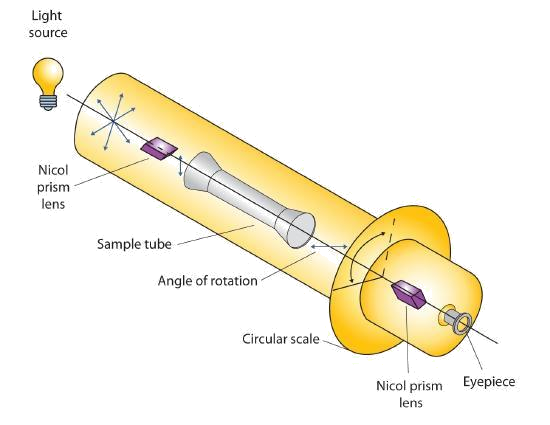
Some optically active substances rotate the plane of polarized light to the right (clockwise) from the observer’s point of view. These compounds are said to be dextrorotatory; substances that rotate light to the left (counterclockwise) are levorotatory. To denote the direction of rotation, a positive sign (+) is given to dextrorotatory substances, and a negative sign (−) is given to levorotatory substances.
Important Hexoses
Although a variety of monosaccharides are found in living organisms, three hexoses are particularly abundant: D-glucose, D-galactose, and D-fructose (Figure 28.1h.). Glucose and galactose are both aldohexoses, while fructose is a ketohexose.
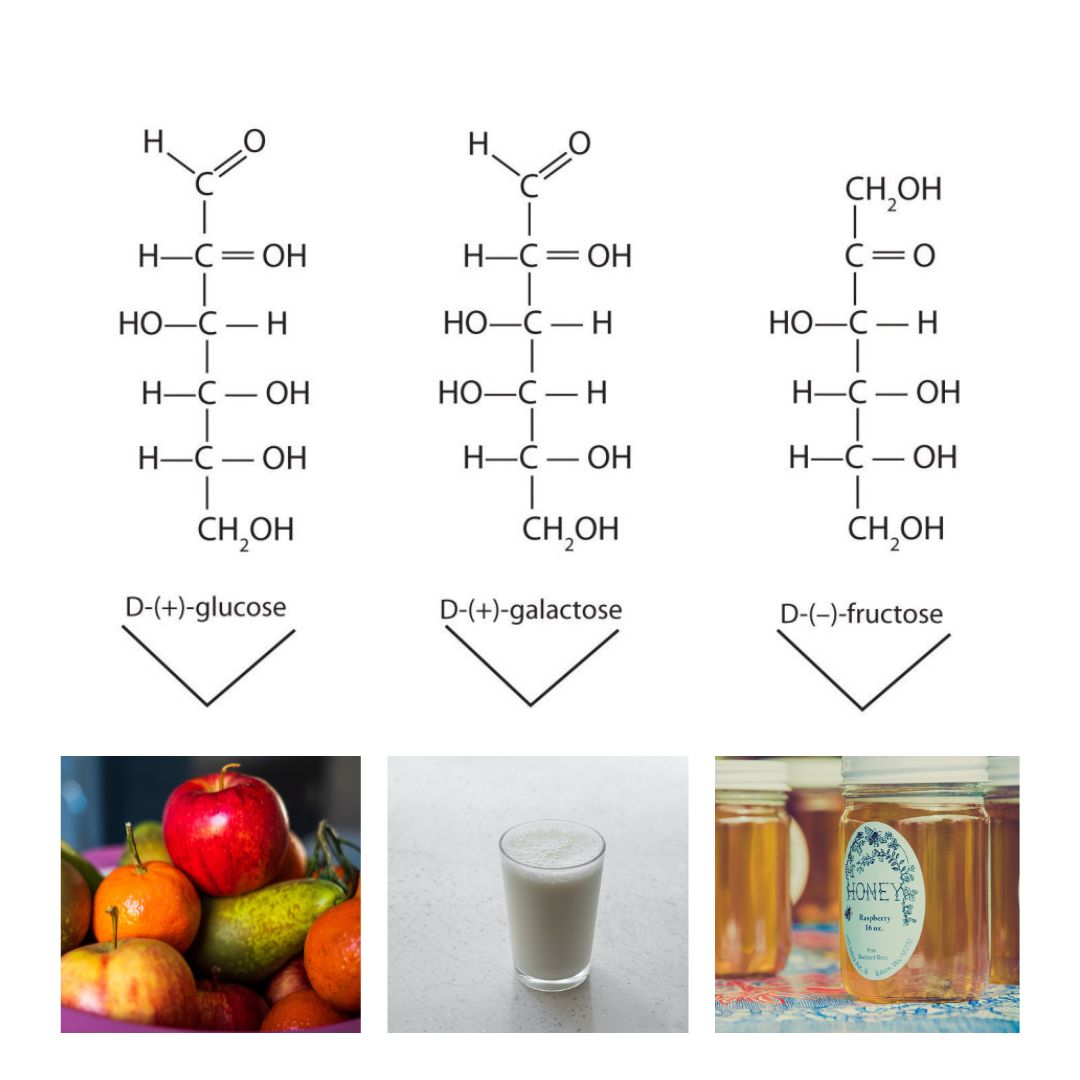
Glucose
D-Glucose, generally referred to as simply glucose, is the most abundant sugar found in nature; most of the carbohydrates we eat are eventually converted to it in a series of biochemical reactions that produce energy for our cells (Figure 28.1i.). It is also known by three other names: dextrose, from the fact that it rotates plane-polarized light in a clockwise (dextrorotatory) direction; corn sugar because in the United States cornstarch is used in the commercial process that produces glucose from the hydrolysis of starch; and blood sugar because it is the carbohydrate found in the circulatory system of animals. Normal blood sugar values range from 70 to 105 mg glucose/dL plasma, and normal urine may contain anywhere from a trace to 20 mg glucose/dL urine.
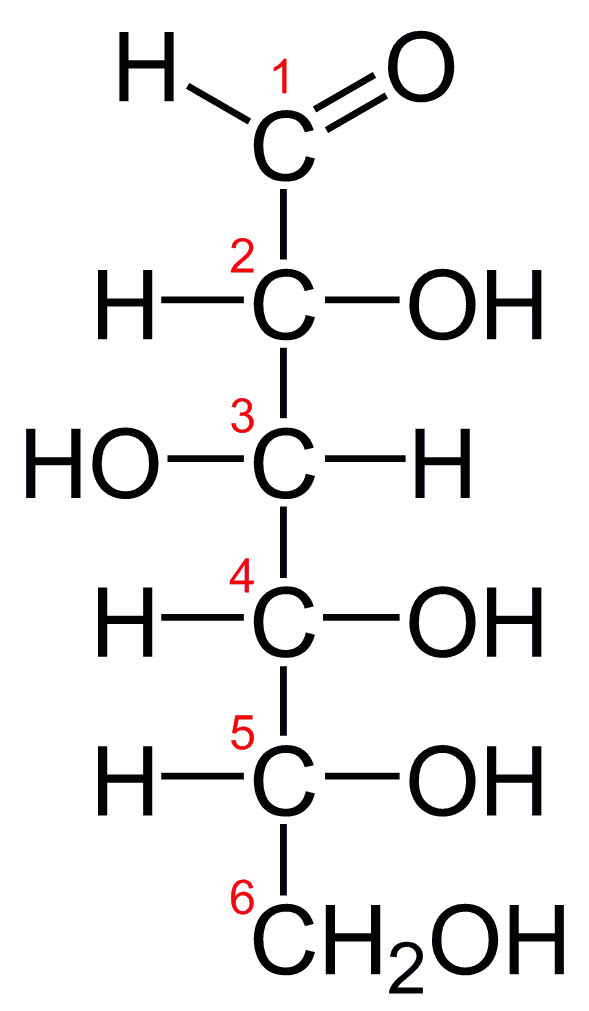
Glucose is a D sugar because the OH group on the fifth carbon atom (the chiral center farthest from the carbonyl group) is on the right. In fact, all the OH groups except the one on the third carbon atom are to the right.
Indigenous Perspectives: Kivak, a Greenland Inuit Delicacy
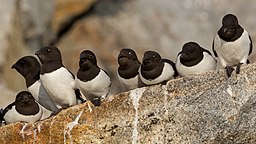
A delicacy within the Inuit is Kivak. It involves the fermentation of glucose using lactic acid bacteria in a process where little auks (Figure 28.1j.) are stuffed into seal skin and stored under rocks for 18 months. This process helps with the preservation and flavours of the dish. For more information see the infographic Compound Interest: The Chemistry Advent Calendar 2023 (compoundchem.com)
Galactose
D-Galactose does not occur in nature in the uncombined state. It is released when lactose, a disaccharide found in milk, is hydrolyzed. The galactose needed by the human body for the synthesis of lactose is obtained by the metabolic conversion of D-glucose to D-galactose. Galactose is also an important constituent of the glycolipids that occur in the brain and the myelin sheath of nerve cells. For this reason it is also known as brain sugar. The structure of D-galactose is shown in Figure 28.1h. Notice that the configuration differs from that of glucose only at the fourth carbon atom.
Fructose
D-Fructose, also shown in Figure 28.1h, is the most abundant ketohexose. Note that from the third through the sixth carbon atoms, its structure is the same as that of glucose. It occurs, along with glucose and sucrose, in honey (which is 40% fructose) and sweet fruits. Fructose (from the Latin fructus, meaning “fruit”) is also referred to as levulose because it has a specific rotation that is strongly levorotatory (−92.4°). It is the sweetest sugar, being 1.7 times sweeter than sucrose, although many nonsugars are several hundred or several thousand times as sweet (Table 28.1a.).
Compound | Relative Sweetness |
---|---|
lactose | 16 |
maltose | 32 |
glucose | 74 |
sucrose | 100 |
fructose | 173 |
aspartame | 18,000 |
acesulfame K | 20,000 |
saccharin | 30,000 |
sucralose | 60,000 |
Source: “16.3: Important Hexoses” In Basics of GOB Chemistry (Ball et al.), CC BY-NC-SA 4.0.
Spotlight on Everyday Chemistry: Artificial Sweeteners
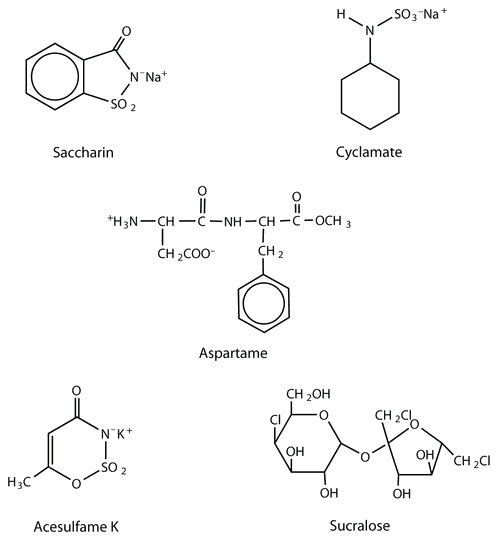
Although sweetness is commonly associated with mono- and disaccharides, it is not a property found only in sugars. Several other kinds of organic compounds have been synthesized that are far superior as sweetening agents. These so-called high-intensity or artificial sweeteners are useful for people with diabetes or other medical conditions that require them to control their carbohydrate intake. The synthetic compounds are noncaloric or used in such small quantities that they do not add significantly to the caloric value of food.
The first artificial sweetener—saccharin—was discovered by accident in 1879. It is 300 times sweeter than sucrose, but it passes through the body unchanged and thus adds no calories to the diet. After its discovery, saccharin was used until it was banned in the early 1900s. However, during the sugar-short years of World War I, the ban was lifted and was not reinstated at the war’s end. One drawback to the use of saccharin is its bitter, metallic aftertaste. The initial solution to this problem was to combine saccharin with cyclamate, a second artificial sweetener discovered in 1937.
In the 1960s and 1970s, several clinical tests with laboratory animals implicated both cyclamate and saccharin as carcinogenic (cancer-causing) substances. The results from the cyclamate tests were completed first, and cyclamate was banned in the United States in 1969. Then a major study was released in Canada in 1977 indicating that saccharin increased the incidence of bladder cancer in rats. The US Food and Drug Administration (FDA) proposed a ban on saccharin that raised immediate public opposition because saccharin was the only artificial sweetener still available. In response, Congress passed the Saccharin Study and Labeling Act in 1977, permitting the use of saccharin as long as any product containing it was labeled with a consumer warning regarding the possible elevation of the risk of bladder cancer. Today this warning is no longer required; moreover, the FDA is currently reviewing the ban on cyclamate, as 75 additional studies and years of usage in other countries, such as Canada, have failed to show that it has any carcinogenic effect.
A third artificial sweetener, aspartame, was discovered in 1965. This white crystalline compound is about 180 times sweeter than sucrose and has no aftertaste. It was approved for use in 1981 and is used to sweeten a wide variety of foods because it blends well with other food flavours. Aspartame is not used in baked goods, however, because it is not heat stable.
In the body (or when heated), aspartame is initially hydrolyzed to three molecules: the amino acids aspartic acid and phenylalanine and an alcohol methanol. Repeated controversy regarding the safety of aspartame arises partly from the fact that the body metabolizes the released methanol to formaldehyde. It should be noted, though, that a glass of tomato juice has six times as much methanol as a similar amount of a diet soda containing aspartame. The only documented risk connected to aspartame use is for individuals with the genetic disease phenylketonuria (PKU); these individuals lack the enzyme needed to metabolize the phenylalanine released when aspartame is broken down by the body. Because of the danger to people with PKU, all products containing aspartame must carry a warning label.
Acesulfame K, discovered just two years after aspartame (1967), was approved for use in the United States in 1988. It is 200 times sweeter than sugar and, unlike aspartame, is heat stable. It has no lingering aftertaste.
One of the newest artificial sweeteners to gain FDA approval (April 1998) for use in the United States is sucralose, a white crystalline solid approximately 600 times sweeter than sucrose. Sucralose is synthesized from sucrose and has three chlorine atoms substituted for three OH groups. It is noncaloric because it passes through the body unchanged. It can be used in baking because it is heat stable.
The structures of these artificial sweeteners can be seen in Figure 28.1k. All of the extensive clinical studies completed to date have indicated that these artificial sweeteners approved for use in the United States are safe for consumption by healthy individuals in moderate amounts.
Cyclic Structures of Monosaccharides
So far, we have represented monosaccharides as linear molecules, but many of them also adopt cyclic structures. This conversion occurs because of the ability of aldehydes and ketones to react with alcohols, as shown in Figure 28.1l.
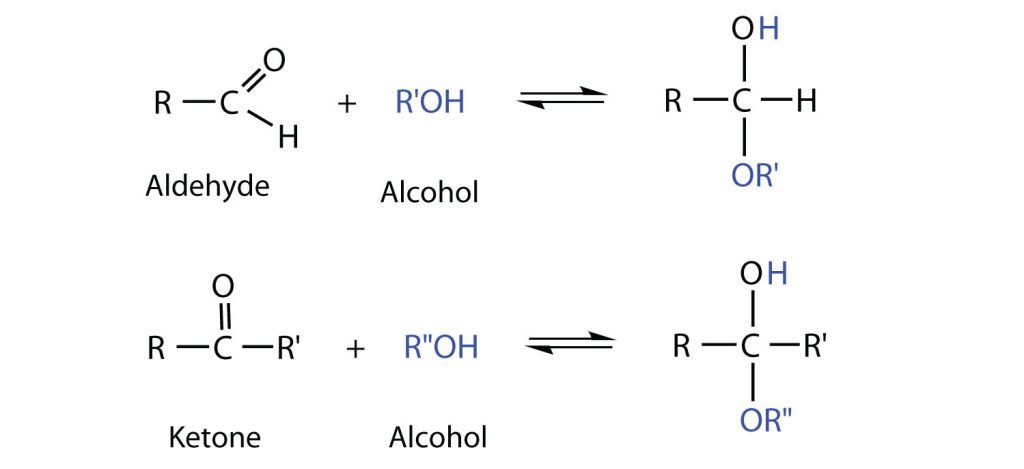
Take a look at the reaction mechanism for how the linear monosaccharide D-glucose adopts a ring structure in Figure 28.1m. You might wonder why the aldehyde reacts with the OH group on the fifth carbon atom rather than the OH group on the second carbon atom next to it. Recall that cyclic alkanes containing five or six carbon atoms in the ring are the most stable. The same is true for monosaccharides that form cyclic structures: rings consisting of five or six carbon atoms are the most stable.
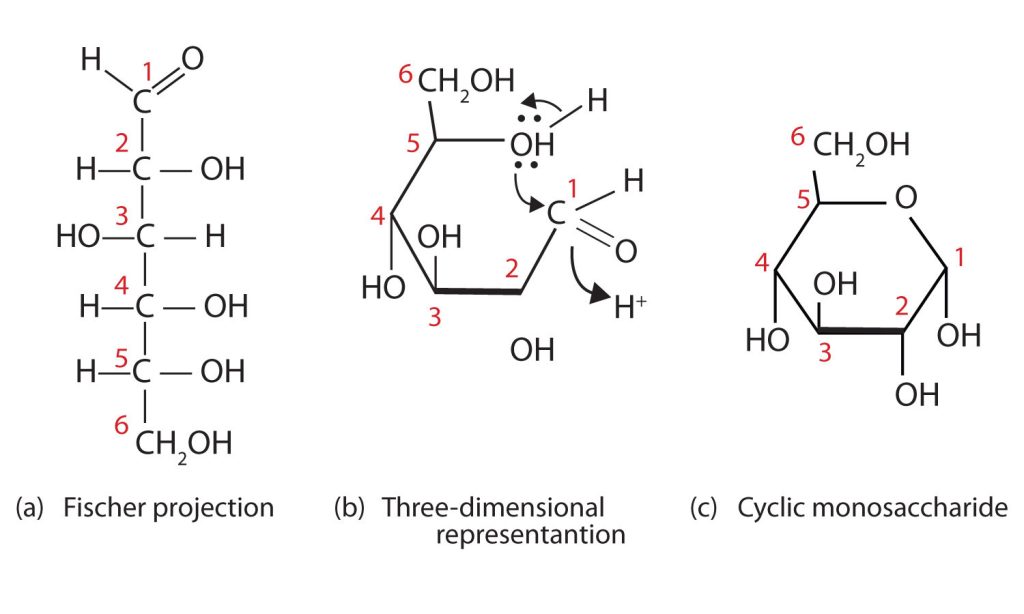
When a straight-chain monosaccharide, such as any of the structures shown in figure 28.1h, forms a cyclic structure, the carbonyl oxygen atom may be pushed either up or down, giving rise to two stereoisomers, as shown in Figure 28.1n. The structure shown on the left side of Figure 28.1n., with the OH group on the first carbon atom projected downward, represent what is called the alpha (α) form. The structures on the right side, with the OH group on the first carbon atom pointed upward, is the beta (β) form. These two stereoisomers of a cyclic monosaccharide are known as anomers; they differ in structure around the anomeric carbon—that is, the carbon atom that was the carbonyl carbon atom in the straight-chain form.
It is possible to obtain a sample of crystalline glucose in which all the molecules have the α structure or all have the β structure. The α form melts at 146°C and has a specific rotation of +112°, while the β form melts at 150°C and has a specific rotation of +18.7°. When the sample is dissolved in water, however, a mixture is soon produced containing both anomers as well as the straight-chain form, in dynamic equilibrium (part (a) of Figure 28.1n.). You can start with a pure crystalline sample of glucose consisting entirely of either anomer, but as soon as the molecules dissolve in water, they open to form the carbonyl group and then reclose to form either the α or the β anomer. The opening and closing repeats continuously in an ongoing interconversion between anomeric forms and is referred to as mutarotation (Latin mutare, meaning “to change”). At equilibrium, the mixture consists of about 36% α-D-glucose, 64% β-D-glucose, and less than 0.02% of the open-chain aldehyde form. The observed rotation of this solution is +52.7°.
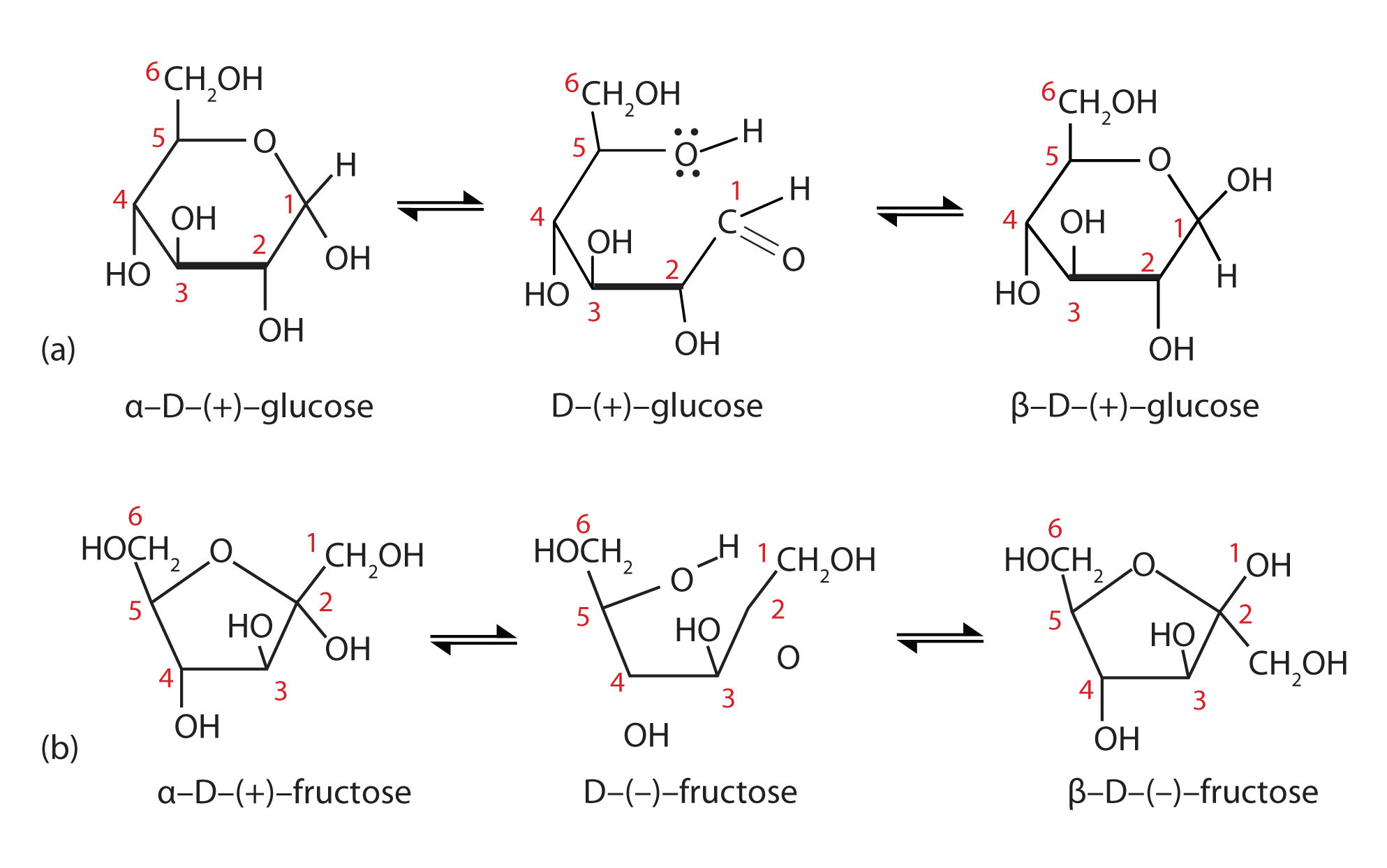
Even though only a small percentage of the molecules are in the open-chain aldehyde form at any time, the solution will nevertheless exhibit the characteristic reactions of an aldehyde. As the small amount of free aldehyde is used up in a reaction, there is a shift in the equilibrium to yield more aldehyde. Thus, all the molecules may eventually react, even though very little free aldehyde is present at a time.
Commonly, the cyclic forms of sugars are depicted using a convention first suggested by Walter N. Haworth, an English chemist. The molecules are drawn as planar hexagons with a darkened edge representing the side facing toward the viewer. The structure is simplified to show only the functional groups attached to the carbon atoms. Any group written to the right in a Fischer projection appears below the plane of the ring in a Haworth projection, and any group written to the left in a Fischer projection appears above the plane in a Haworth projection.
The difference between the α and the β forms of sugars may seem trivial, but such structural differences are often crucial in biochemical reactions. This explains why we can get energy from the starch in potatoes and other plants but not from cellulose, even though both starch and cellulose are polysaccharides composed of glucose molecules linked together.
Chemical Properties of Monosaccharides
Monosaccharides such as glucose and fructose are crystalline solids at room temperature, but they are quite soluble in water, each molecule having several OH groups that readily engage in hydrogen bonding. The chemical behavior of these monosaccharides is likewise determined by their functional groups.
An important reaction of monosaccharides is the oxidation of the aldehyde group, one of the most easily oxidized organic functional groups. Aldehyde oxidation can be accomplished with any mild oxidizing agent, such as Tollens’ reagent or Benedict’s reagent. With the latter, complexed copper(II) ions are reduced to copper(I) ions that form a brick-red precipitate [copper(I) oxide; Figure 28.1o.].
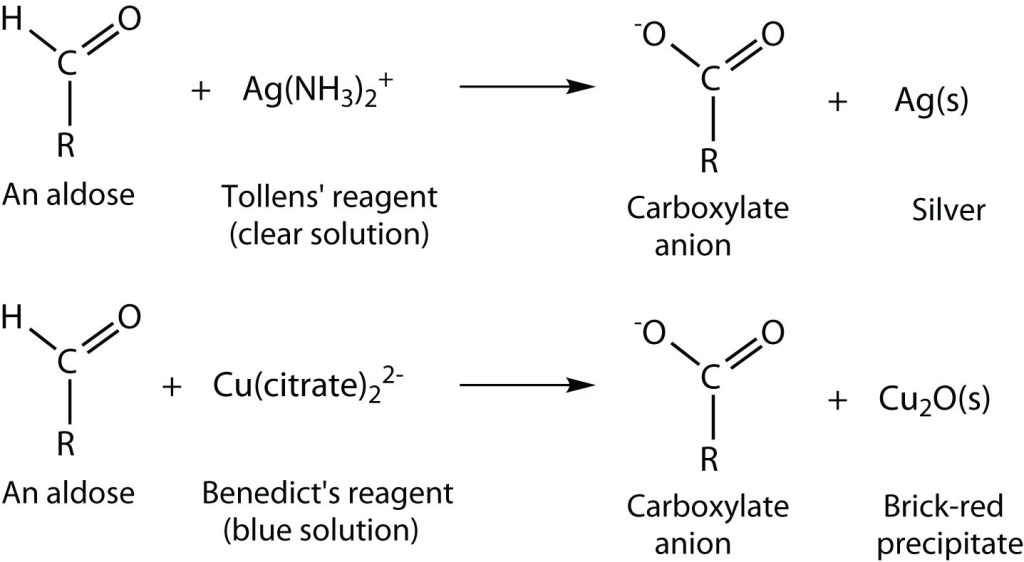
Any carbohydrate capable of reducing either Tollens’ or Benedict’s reagents without first undergoing hydrolysis is said to be a reducing sugar (Figure 28.1p.). Because both the Tollens’ and Benedict’s reagents are basic solutions, ketoses (such as fructose) also give positive tests due to an equilibrium that exists between ketoses and aldoses in a reaction known as tautomerism.
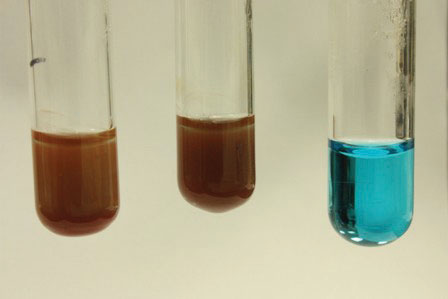
These reactions have been used as simple and rapid diagnostic tests for the presence of glucose in blood or urine. For example, Clinitest tablets, which are used to test for sugar in the urine, contain copper(II) ions and are based on Benedict’s test. A green colour indicates very little sugar, whereas a brick-red colour indicates sugar in excess of 2 g/100 mL of urine.
Disaccharides
Previously, you learned that monosaccharides can form cyclic structures by the reaction of the carbonyl group with an OH group. These cyclic molecules can in turn react with another alcohol. Disaccharides (C12H22O11) are sugars composed of two monosaccharide units that are joined by a carbon–oxygen-carbon linkage known as a glycosidic linkage. This linkage is formed from the reaction of the anomeric carbon of one cyclic monosaccharide with the OH group of a second monosaccharide, as shown in Figure 28.1q.
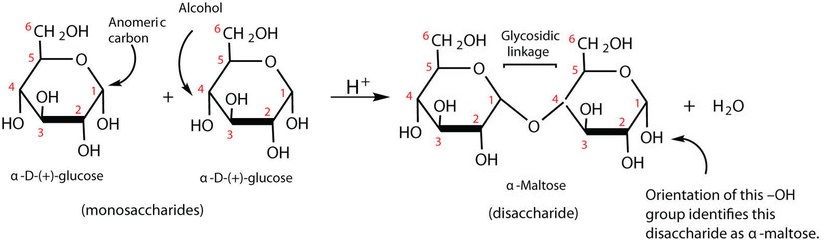
The disaccharides differ from one another in their monosaccharide constituents and in the specific type of glycosidic linkage connecting them. There are three common disaccharides: maltose, lactose, and sucrose. All three are white crystalline solids at room temperature and are soluble in water. We’ll consider each sugar in more detail.
Maltose
Maltose occurs to a limited extent in sprouting grain. It is formed most often by the partial hydrolysis of starch and glycogen. In the manufacture of beer, maltose is liberated by the action of malt (germinating barley) on starch; for this reason, it is often referred to as malt sugar. Maltose is about 30% as sweet as sucrose. The human body is unable to metabolize maltose or any other disaccharide directly from the diet because the molecules are too large to pass through the cell membranes of the intestinal wall. Therefore, an ingested disaccharide must first be broken down by hydrolysis into its two constituent monosaccharide units.
In the body, such hydrolysis reactions are catalyzed by enzymes such as maltase. The same reactions can be carried out in the laboratory with dilute acid as a catalyst, although in that case the rate is much slower, and high temperatures are required. Whether it occurs in the body or a glass beaker, the hydrolysis of maltose produces two molecules of D-glucose:
[latex]\mathrm{maltose \xrightarrow{H^+\: or\: maltase} \textrm{2 D-glucose}} \nonumber[/latex]
Maltose is a reducing sugar. Thus, its two glucose molecules must be linked in such a way as to leave one anomeric carbon that can open to form an aldehyde group. The glucose units in maltose are joined in a head-to-tail fashion through an α-linkage from the first carbon atom of one glucose molecule to the fourth carbon atom of the second glucose molecule (that is, an α-1,4-glycosidic linkage; see Figure 28.1q.). The bond from the anomeric carbon of the first monosaccharide unit is directed downward, which is why this is known as an α-glycosidic linkage. The OH group on the anomeric carbon of the second glucose can be in either the α or the β position, as shown in Figure 28.1r.
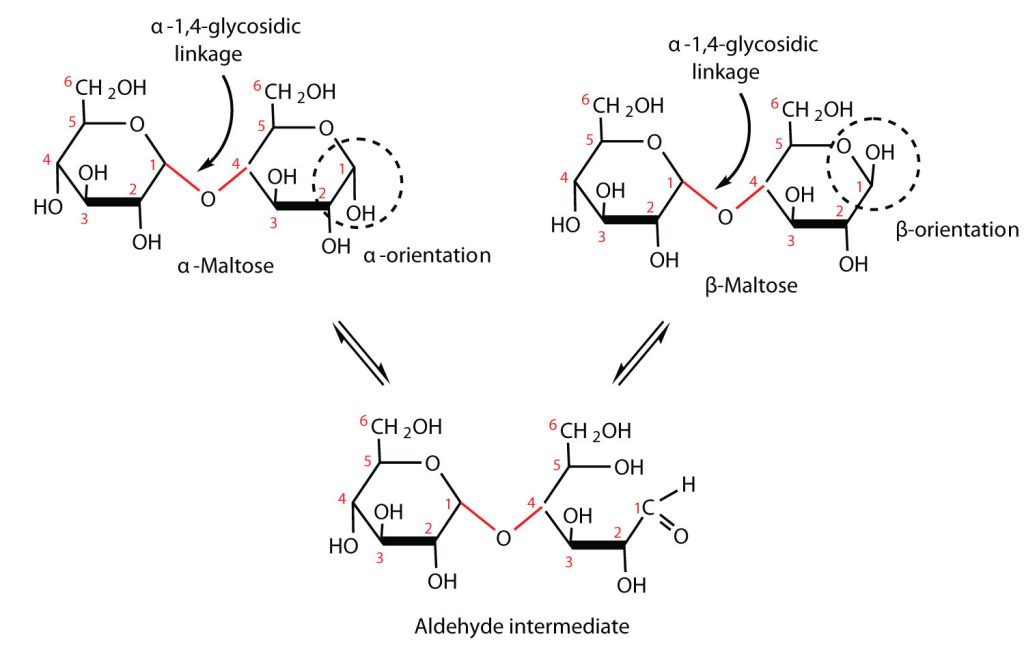
Lactose
Lactose is known as milk sugar because it occurs in the milk of humans, cows, and other mammals. In fact, the natural synthesis of lactose occurs only in mammary tissue, whereas most other carbohydrates are plant products. Human milk contains about 7.5% lactose, and cow’s milk contains about 4.5%. This sugar is one of the lowest ranking in terms of sweetness, being about one-sixth as sweet as sucrose. Lactose is produced commercially from whey, a by-product in the manufacture of cheese. It is important as an infant food and in the production of penicillin.
Lactose is a reducing sugar composed of one molecule of D-galactose and one molecule of D-glucose joined by a β-1,4-glycosidic bond (the bond from the anomeric carbon of the first monosaccharide unit being directed upward). The two monosaccharides are obtained from lactose by acid hydrolysis or the catalytic action of the enzyme lactase, as shown in Figure 28.1s.
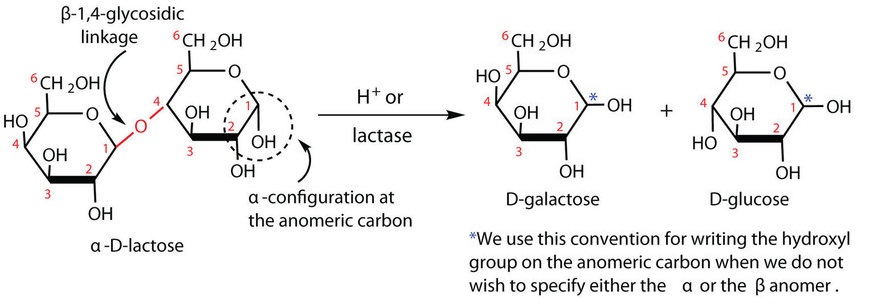
Many adults and some children suffer from a deficiency of lactase. These individuals are said to be lactose intolerant because they cannot digest the lactose found in milk. A more serious problem is the genetic disease galactosemia, which results from the absence of an enzyme needed to convert galactose to glucose. Certain bacteria can metabolize lactose, forming lactic acid as one of the products. This reaction is responsible for the “souring” of milk.
For this trisaccharide, indicate whether each glycosidic linkage is α or β.
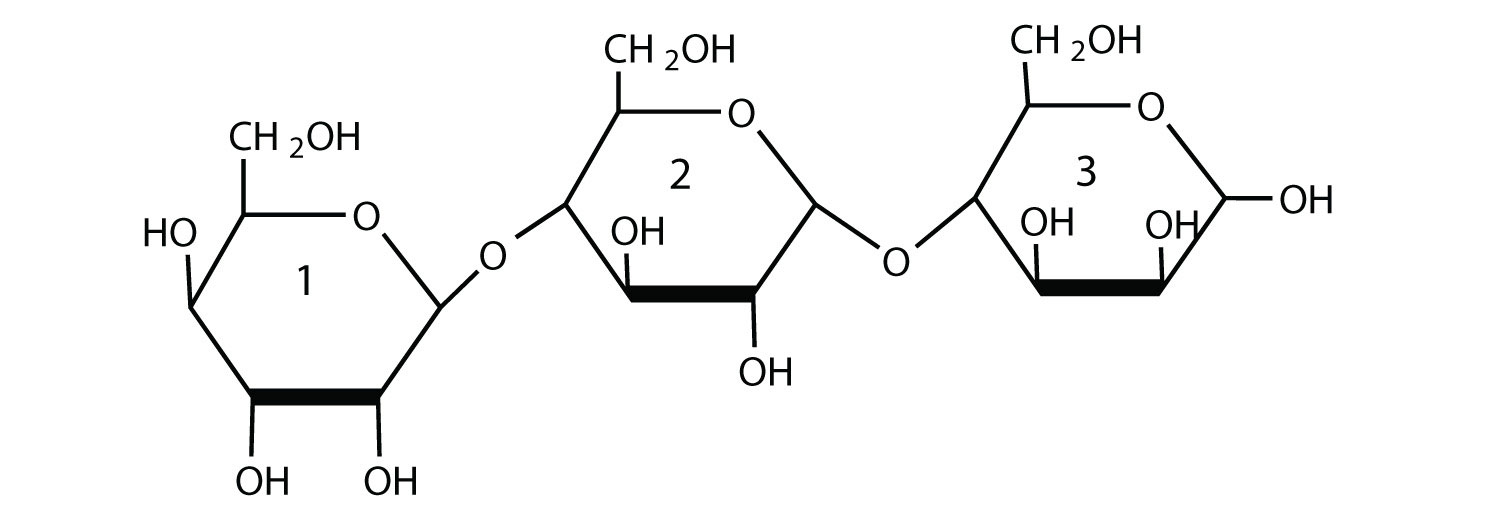
Solution
The glycosidic linkage between sugars 1 and 2 is β because the bond is directed up from the anomeric carbon. The glycosidic linkage between sugars 2 and 3 is α because the bond is directed down from the anomeric carbon.
For this trisaccharide, indicate whether each glycosidic linkage is α or β.
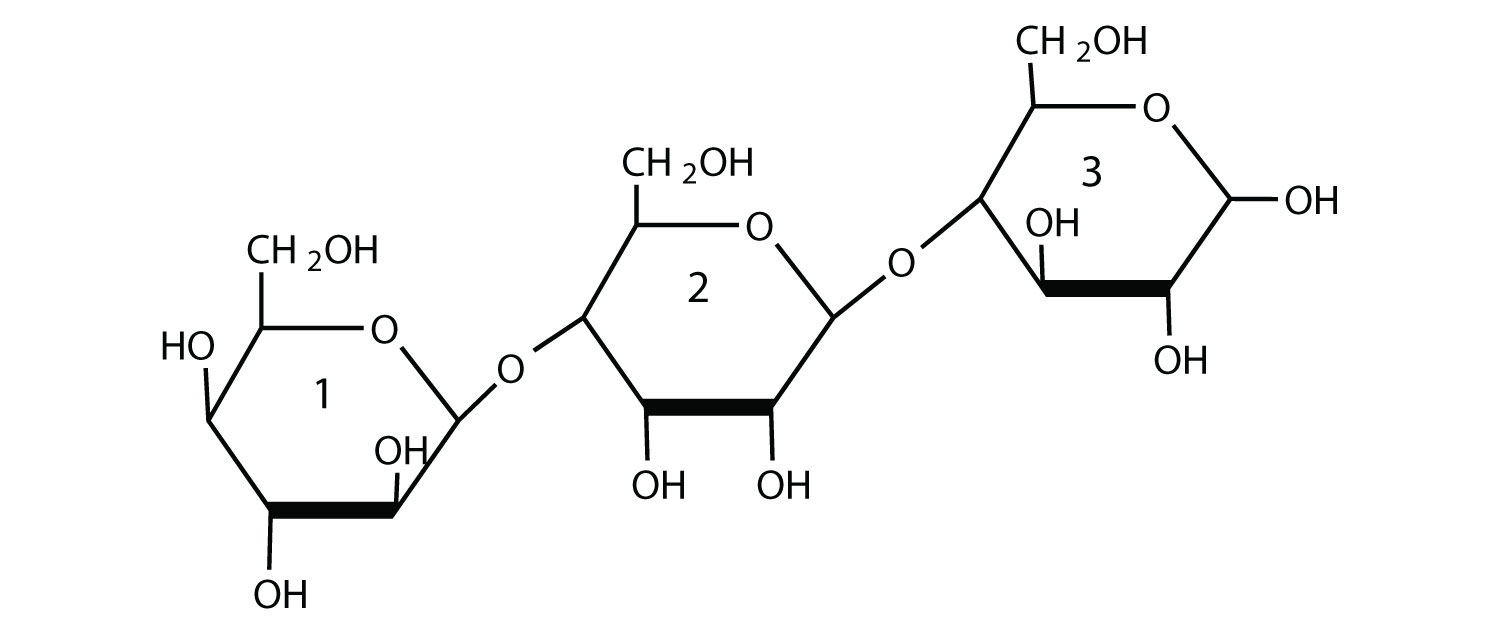
Check Your Answer[2]
Spotlight on Everyday Chemistry: Lactose Intolerance and Galactosemia
Lactose makes up about 40% of an infant’s diet during the first year of life. Infants and small children have one form of the enzyme lactase in their small intestines and can digest the sugar easily; however, adults usually have a less active form of the enzyme, and about 70% of the world’s adult population has some deficiency in its production. As a result, many adults experience a reduction in the ability to hydrolyze lactose to galactose and glucose in their small intestine. For some people the inability to synthesize sufficient enzyme increases with age. Up to 20% of the US population suffers some degree of lactose intolerance.
In people with lactose intolerance, some of the unhydrolyzed lactose passes into the colon, where it tends to draw water from the interstitial fluid into the intestinal lumen by osmosis. At the same time, intestinal bacteria may act on the lactose to produce organic acids and gases. The buildup of water and bacterial decay products leads to abdominal distention, cramps, and diarrhea, which are symptoms of the condition.
The symptoms disappear if milk or other sources of lactose are excluded from the diet or consumed only sparingly. Alternatively, many food stores now carry special brands of milk that have been pretreated with lactase to hydrolyze the lactose. Cooking or fermenting milk causes at least partial hydrolysis of the lactose, so some people with lactose intolerance are still able to enjoy cheese, yogurt, or cooked foods containing milk. The most common treatment for lactose intolerance, however, is the use of lactase preparations (e.g., Lactaid), which are available in liquid and tablet form at drugstores and grocery stores. These are taken orally with dairy foods—or may be added to them directly—to assist in their digestion.
Galactosemia is a condition in which one of the enzymes needed to convert galactose to glucose is missing. Consequently, the blood galactose level is markedly elevated, and galactose is found in the urine. An infant with galactosemia experiences a lack of appetite, weight loss, diarrhea, and jaundice. The disease may result in impaired liver function, cataracts, mental retardation, and even death. If galactosemia is recognized in early infancy, its effects can be prevented by the exclusion of milk and all other sources of galactose from the diet. As a child with galactosemia grows older, he or she usually develops an alternate pathway for metabolizing galactose, so the need to restrict milk is not permanent. The incidence of galactosemia in the United States is 1 in every 65,000 newborn babies.
Sucrose
Sucrose, probably the largest-selling pure organic compound in the world, is known as beet sugar, cane sugar, table sugar, or simply sugar. Most of the sucrose sold commercially is obtained from sugar cane and sugar beets (whose juices are 14%–20% sucrose) by evaporation of the water and recrystallization. The dark brown liquid that remains after the recrystallization of sugar is sold as molasses.
The sucrose molecule is unique among the common disaccharides in having an α-1,β-2-glycosidic (head-to-head) linkage. Because this glycosidic linkage is formed by the OH group on the anomeric carbon of α-D-glucose and the OH group on the anomeric carbon of β-D-fructose, it ties up the anomeric carbons of both glucose and fructose, as shown in Figure 28.1t.
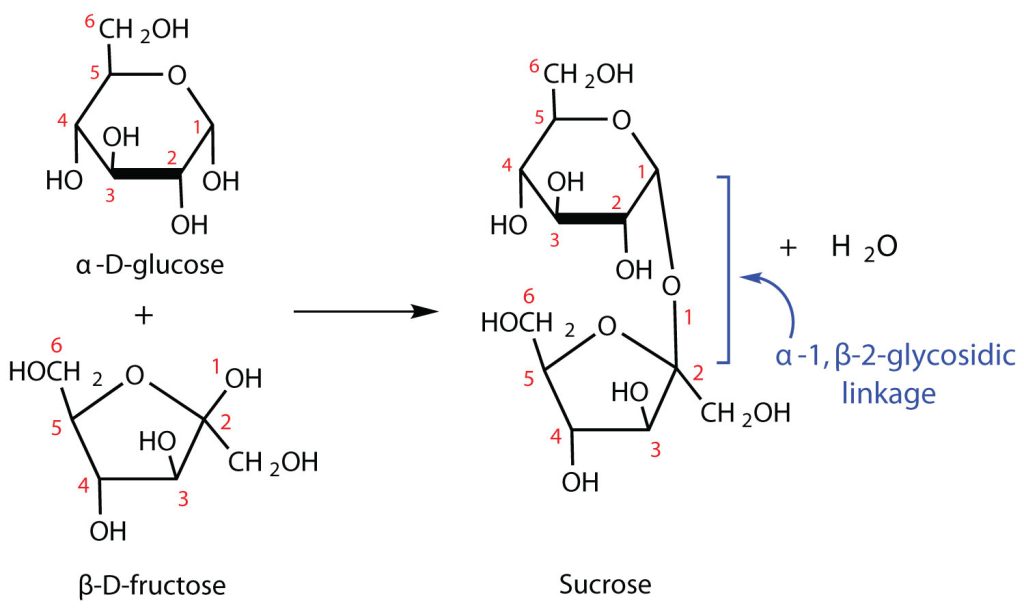
This linkage gives sucrose certain properties that are quite different from those of maltose and lactose. As long as the sucrose molecule remains intact, neither monosaccharide “uncyclizes” to form an open-chain structure. Thus, sucrose is incapable of mutarotation and exists in only one form both in the solid state and in solution. In addition, sucrose does not undergo reactions that are typical of aldehydes and ketones. Therefore, sucrose is a nonreducing sugar.
The hydrolysis of sucrose in dilute acid or through the action of the enzyme sucrase (also known as invertase) gives an equimolar mixture of glucose and fructose. This 1:1 mixture is referred to as invert sugar because it rotates plane-polarized light in the opposite direction than sucrose. The hydrolysis reaction has several practical applications. Sucrose readily recrystallizes from a solution, but invert sugar has a much greater tendency to remain in solution. In the manufacture of jelly and candy and in the canning of fruit, the recrystallization of sugar is undesirable. Therefore, conditions leading to the hydrolysis of sucrose are employed in these processes. Moreover, because fructose is sweeter than sucrose, the hydrolysis adds to the sweetening effect. Bees carry out this reaction when they make honey.
The average American consumes more than 100 lb of sucrose every year. About two-thirds of this amount is ingested in soft drinks, presweetened cereals, and other highly processed foods. The widespread use of sucrose is a contributing factor to obesity and tooth decay. Carbohydrates such as sucrose, are converted to fat when the caloric intake exceeds the body’s requirements, and sucrose causes tooth decay by promoting the formation of plaque that sticks to teeth.
Polysaccharides
The polysaccharides are the most abundant carbohydrates in nature and serve a variety of functions, such as energy storage or as components of plant cell walls. Polysaccharides are very large polymers composed of tens to thousands of monosaccharides joined together by glycosidic linkages. The three most abundant polysaccharides are starch, glycogen, and cellulose. These three are referred to as homopolymers because each yields only one type of monosaccharide (glucose) after complete hydrolysis. Heteropolymers may contain sugar acids, amino sugars, or noncarbohydrate substances in addition to monosaccharides. Heteropolymers are common in nature (gums, pectins, and other substances) but will not be discussed further in this textbook. The polysaccharides are nonreducing carbohydrates, are not sweet tasting, and do not undergo mutarotation.
Starch
Starch is the most important source of carbohydrates in the human diet and accounts for more than 50% of our carbohydrate intake. It occurs in plants in the form of granules, and these are particularly abundant in seeds (especially the cereal grains) and tubers, where they serve as a storage form of carbohydrates. The breakdown of starch to glucose nourishes the plant during periods of reduced photosynthetic activity. We often think of potatoes as a “starchy” food, yet other plants contain a much greater percentage of starch (potatoes 15%, wheat 55%, corn 65%, and rice 75%). Commercial starch is a white powder.
Starch is a mixture of two polymers: amylose and amylopectin. Natural starches consist of about 10%–30% amylose and 70%–90% amylopectin. Amylose is a linear polysaccharide composed entirely of D-glucose units joined by the α-1,4-glycosidic linkages we saw in maltose (part (a) of Figure 28.1u.). Experimental evidence indicates that amylose is not a straight chain of glucose units but instead is coiled like a spring, with six glucose monomers per turn (part (b) of Figure 28.1u.). When coiled in this fashion, amylose has just enough room in its core to accommodate an iodine molecule. The characteristic blue-violet colour that appears when starch is treated with iodine is due to the formation of the amylose-iodine complex. This colour test is sensitive enough to detect even minute amounts of starch in solution.
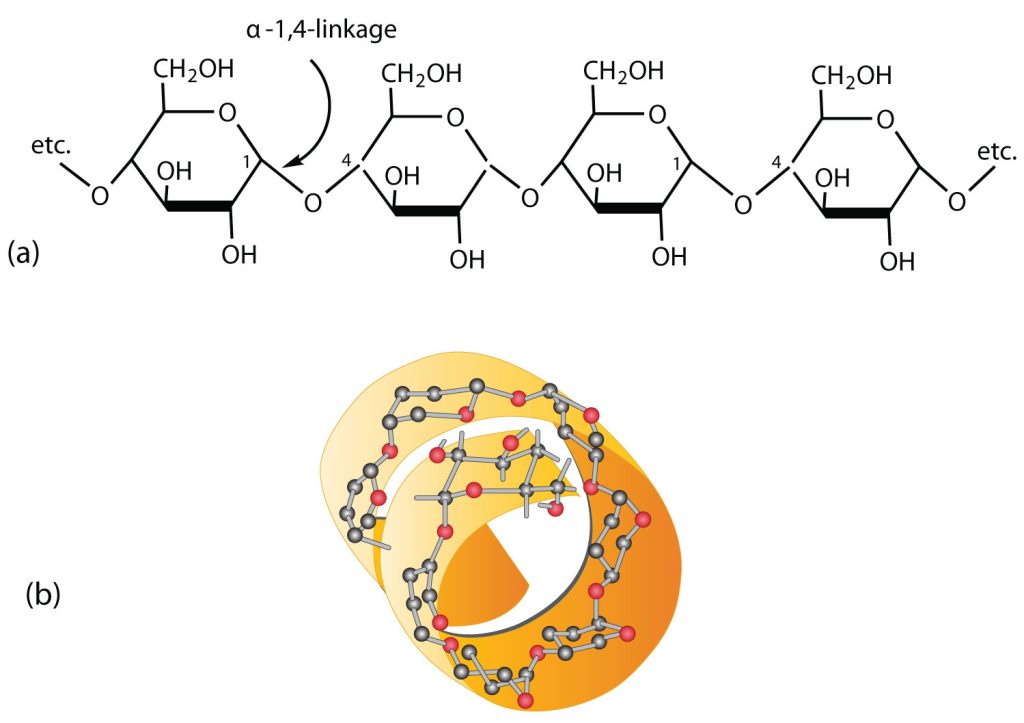
Amylopectin is a branched-chain polysaccharide composed of glucose units linked primarily by α-1,4-glycosidic bonds but with occasional α-1,6-glycosidic bonds, which are responsible for the branching. A molecule of amylopectin may contain many thousands of glucose units with branch points occurring about every 25–30 units (Figure 28.1v.). The helical structure of amylopectin is disrupted by the branching of the chain, so instead of the deep blue-violet colour amylose gives with iodine, amylopectin produces a less intense reddish brown.
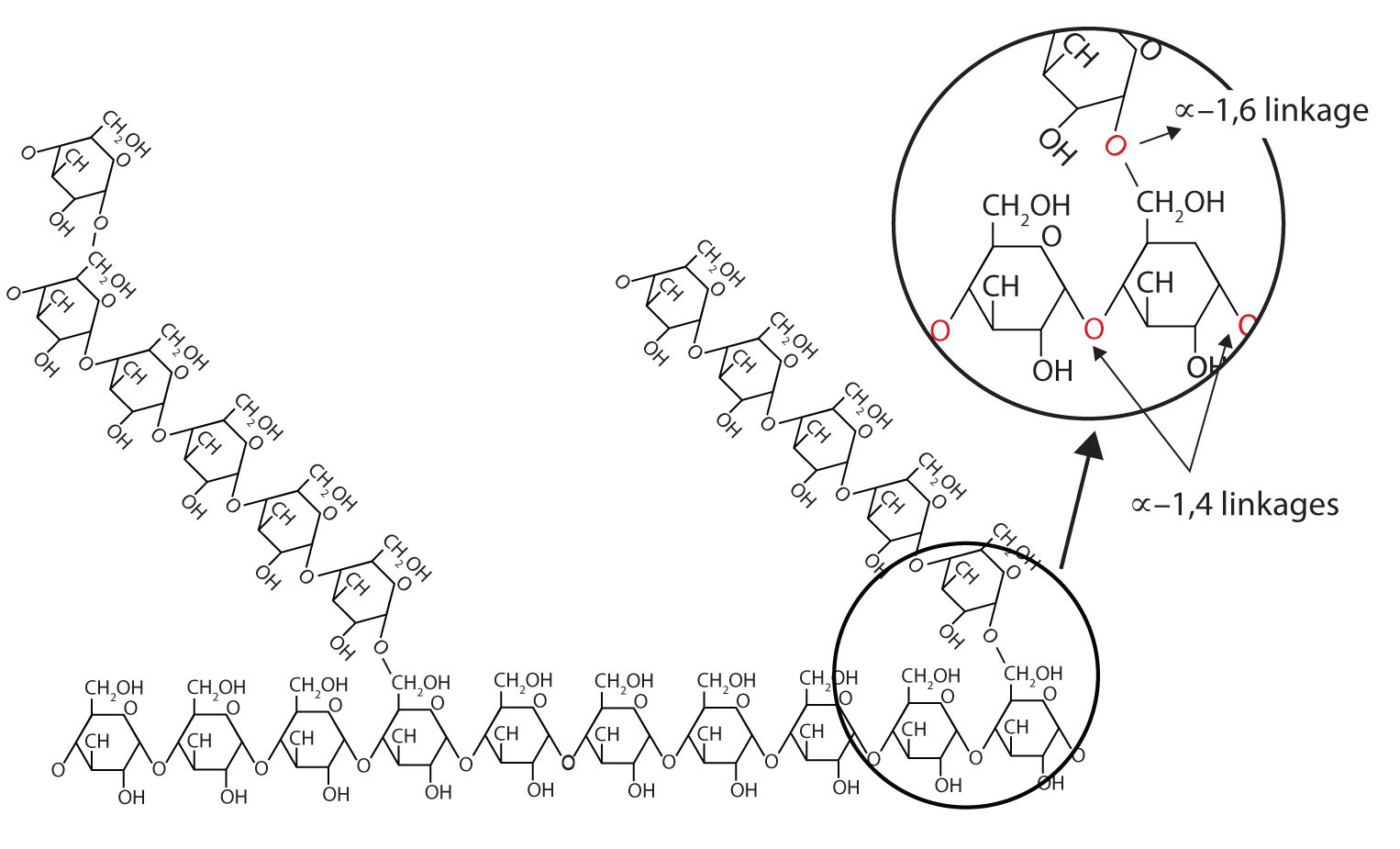
Dextrins are glucose polysaccharides of intermediate size. The shine and stiffness imparted to clothing by starch are due to the presence of dextrins formed when clothing is ironed. Because of their characteristic stickiness with wetting, dextrins are used as adhesives on stamps, envelopes, and labels; as binders to hold pills and tablets together; and as pastes. Dextrins are more easily digested than starch and are therefore used extensively in the commercial preparation of infant foods.
The complete hydrolysis of starch yields, in successive stages, glucose:
starch → dextrins → maltose → glucose
In the human body, several enzymes known collectively as amylases degrade starch sequentially into usable glucose units.
Glycogen
Glycogen is the energy reserve carbohydrate of animals. Practically all mammalian cells contain some stored carbohydrates in the form of glycogen, but it is especially abundant in the liver (4%–8% by weight of tissue) and in skeletal muscle cells (0.5%–1.0%). Like starch in plants, glycogen is found as granules in liver and muscle cells. When fasting, animals draw on these glycogen reserves during the first day without food to obtain the glucose needed to maintain metabolic balance.
Glycogen is structurally quite similar to amylopectin, although glycogen is more highly branched (8–12 glucose units between branches) and the branches are shorter. When treated with iodine, glycogen gives a reddish brown colour. Glycogen can be broken down into its D-glucose subunits by acid hydrolysis or by the same enzymes that catalyze the breakdown of starch. In animals, the enzyme phosphorylase catalyzes the breakdown of glycogen to phosphate esters of glucose. About 70% of the total glycogen in the body is stored in muscle cells. Although the percentage of glycogen (by weight) is higher in the liver, the much greater mass of skeletal muscle stores a greater total amount of glycogen.
Cellulose
Cellulose, a fibrous carbohydrate found in all plants, is the structural component of plant cell walls. Because the earth is covered with vegetation, cellulose is the most abundant of all carbohydrates, accounting for over 50% of all the carbon found in the vegetable kingdom. Cotton fibrils and filter paper are almost entirely cellulose (about 95%), wood is about 50% cellulose, and the dry weight of leaves is about 10%–20% cellulose. The largest use of cellulose is in the manufacture of paper and paper products. Although the use of noncellulose synthetic fibers is increasing, rayon (made from cellulose) and cotton still account for over 70% of textile production.
Like amylose, cellulose is a linear polymer of glucose. It differs, however, in that the glucose units are joined by β-1,4-glycosidic linkages, producing a more extended structure than amylose (part (a) of Figure 28.1w.). This extreme linearity allows a great deal of hydrogen bonding between OH groups on adjacent chains, causing them to pack closely into fibers (part (b) of Figure 28.1w.). As a result, cellulose exhibits little interaction with water or any other solvent. Cotton and wood, for example, are completely insoluble in water and have considerable mechanical strength. Because cellulose does not have a helical structure, it does not bind to iodine to form a coloured product.
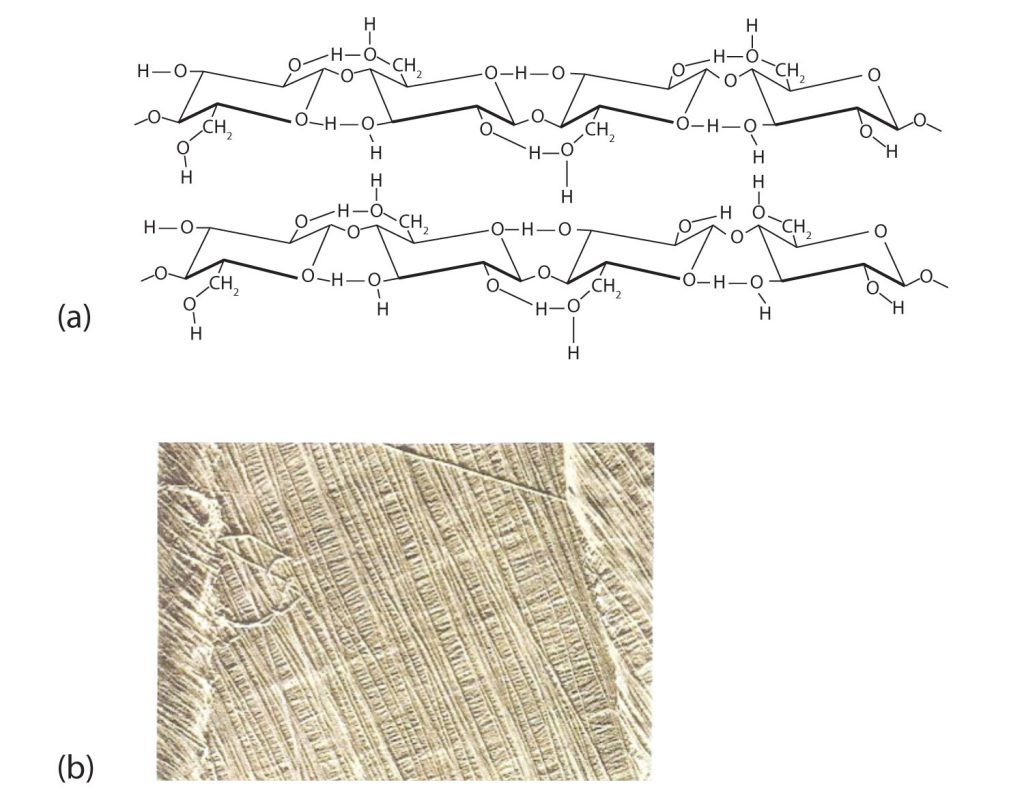
Cellulose yields D-glucose after complete acid hydrolysis, yet humans are unable to metabolize cellulose as a source of glucose. Our digestive juices lack enzymes that can hydrolyze the β-glycosidic linkages found in cellulose, so although we can eat potatoes, we cannot eat grass. However, certain microorganisms can digest cellulose because they make the enzyme cellulase, which catalyzes the hydrolysis of cellulose. The presence of these microorganisms in the digestive tracts of herbivorous animals (such as cows, horses, and sheep) allows these animals to degrade the cellulose from plant material into glucose for energy. Termites also contain cellulase-secreting microorganisms and thus can subsist on a wood diet. This example once again demonstrates the extreme stereospecificity of biochemical processes.
Attribution & References
Except where otherwise noted, this page is adapted by Gregory A. Anderson and Samantha Sullivan Sauer from “16: Carbohydrates” Basics of General, Organic, and Biological Chemistry (Ball et al.) by David W. Ball, John W. Hill, and Rhonda J. Scott via LibreTexts, CC BY-NC-SA 4.0./ A LibreTexts version of Introduction to Chemistry: GOB (v. 1.0), CC BY-NC 3.0. Content has been condensed into one page, edited for student understanding and enhanced to match this OER.
-
Glucose has an aldehyde functional group and multiple alcohol functional groups making it polar and soluble in water. Fructose has a ketone functional group and multiple alcohol functional groups making it polar and soluble in water.
↵
-
The glycosidic linkage between sugars 1 and 2 and the linkage between sugars 2 and 3 are both β because the bonds are directed up from the anomeric carbon in each case.
↵
isomers having the same structural formula but differing in the arrangement of atoms or groups of atoms in three-dimensional space
Molecules that are nonsuperimposable (nonidentical) mirror images of each other
the aldehyde group is written at the top, and the hydrogen atoms and OH groups that are attached to each chiral carbon are written to the right or left. (If the monosaccharide is a ketose, the ketone functional group is the second carbon atom.) Vertical lines represent bonds pointing away from you, while horizontal lines represent bonds coming toward you.
optically active substances that rotate the plane of polarized light to the right (clockwise) from the observer’s point of view
optically active substances rotate the plane of polarized light to the left (counterclockwise) from the observer’s point of view