8. Microbial Catabolism
8.3 Cellular Respiration
Learning Objectives
- Compare and contrast the electron transport system location and function in a prokaryotic cell and a eukaryotic cell
- Compare and contrast the differences between substrate-level and oxidative phosphorylation
- Explain the relationship between chemiosmosis and proton motive force
- Describe the function and location of ATP synthase in a prokaryotic versus eukaryotic cell
- Describe various types of electron acceptors in anaerobic respiration and compare to aerobic respiration
We have just discussed two pathways in glucose catabolism—glycolysis and the Krebs cycle—that generate ATP by substrate-level phosphorylation. Most ATP, however, is generated during a separate process called oxidative phosphorylation, which occurs during cellular respiration. Cellular respiration occurs in chemotrophs: lithotrophs and heterotrophs. It begins when electrons are transferred from the lithotrophic energy source, or in heterotrophs, from NADH and FADH2 (made in glycolysis, the transition reaction, and the Krebs cycle), through an electron transport chain. This chain catalyzes a series of chemical reactions, where each component of the chain is successively reduced, then oxidized, finally being donated to a terminal inorganic electron acceptor. This electron acceptor is either oxygen in aerobic respiration or, in anaerobic bacteria and archaea, some other inorganic molecule (Figure 8.19). These electron transfers take place in the plasma membrane of bacteria and archaea, or in specialized protein complexes in the inner membrane of the mitochondria of eukaryotic cells. The energy of the electrons is harvested to generate an electrochemical gradient across the membrane, which is used to make ATP by oxidative phosphorylation.
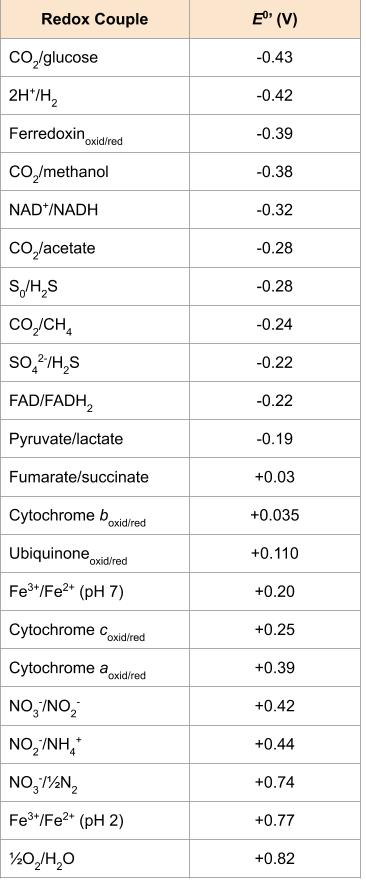
Electron Transport System
The electron transport system (ETS) comprises a series of membrane-associated protein complexes and associated mobile accessory electron carriers. In bacteria and archaea, this is in the plasma membrane; in eukaryotes, this is in the mitochondrion (phototrophic electron transport systems are very similar, and are discussed later in this chapter). Electron transport is a series of chemical reactions that resembles a bucket brigade in that electrons are passed rapidly from one ETS electron carrier to the next. In heterotrophs, electrons enter the system through the reduced NADH and FADH2 cofactors from the central pathways, or an external or intracellular inorganic energy source in the lithotrophs.
While there are many different electron carriers, some unique to specific organisms or groups of organisms, some of the more common ones are listed below and also in Figure 8.19. Whether a particular ETS component accepts electrons and protons, or just electrons is critical to chemiosmosis and energy generation, as discussed further below.
- Nicotinamide adenine dinucleotide (NAD+/NADH) – a co-enzyme that carriers both electrons (e–) and protons (H+), two of each. A closely related molecule is nicotinamide adenine dinucleotide phosphate (NADP+/ NADPH), which accepts 2 electrons and 1 proton.
- Flavin adenine dinucleotide (FAD/FADH2) and flavin mononucleotide (FMN/FMNH2) – carry 2 electrons and 2 protons each. Proteins with these molecules are called flavoproteins.
- Coenzyme Q (CoQ)/ubiquinone – a small, lipid soluble ETS component that alternates from quinone to quinol upon reduction by 2 electrons and 2 protons.
- Cytochromes – immobile ETS components that use iron atoms as part of a heme group, carrying 1 electron at a time with reduction of ferric iron (Fe3+) to ferrous iron (Fe2+).
- Iron-sulphur (Fe-S) proteins, such as ferredoxin – immobile ETS components that use iron atoms not part of heme group to carry 1 electron at a time.
ETS carriers are arranged so that electrons move spontaneously, based on their redox potential, from those with more negative redox potential (i.e. higher in the tower) to those with more positive redox potential.
Chemiosmosis, Proton Motive Force, and Oxidative Phosphorylation
In each transfer of an electron through the ETS, the electron loses energy, but with some transfers, the energy is stored as potential energy by using it to pump hydrogen ions (H+) across a membrane. In prokaryotic cells, H+ is pumped to the outside of the cytoplasmic membrane (i.e. the periplasmic space in gram-negative and gram-positive bacteria), and in eukaryotic cells, they are pumped from the mitochondrial matrix across the inner mitochondrial membrane into the intermembrane space. There is an uneven distribution of H+ across the membrane that establishes an electrochemical gradient because of the greater density of positively charged H+ ions (ΔΨ) and the higher chemical concentration (i.e.ΔpH) on one side of the membrane. This electrochemical gradient is the proton motive force (Δp or PMF). Beyond the use of the PMF to make ATP, as discussed in this chapter, the PMF can also be used to drive other energetically unfavourable processes, including nutrient transport and flagellar rotation for motility.
The energy in the proton motive force is converted to ATP through a process called chemiosmosis. The protons diffuse across the membrane (the plasma membrane in prokaryotic cells and the inner membrane in mitochondria in eukaryotic cells), following their concentration gradient, through the membrane-bound ATP synthase complex (Figure 8.20). The tendency for movement in this way is much like water accumulated on one side of a dam, moving through the dam when opened. ATP synthase (like a combination of the intake and generator of a hydroelectric dam) is a complex protein that acts as a tiny generator, turning by the force of the H+ diffusing through the enzyme, down their electrochemical gradient from where there are many mutually repelling H+ to where there are fewer H+. In prokaryotic cells, H+ flows from the outside of the cytoplasmic membrane into the cytoplasm, whereas in eukaryotic mitochondria, H+ flows from the intermembrane space to the mitochondrial matrix. The turning of the parts of this molecular machine regenerates ATP from ADP and inorganic phosphate (Pi) by oxidative phosphorylation, a second mechanism for making ATP that harvests the potential energy stored within an electrochemical gradient.

Aerobic Respiration
In aerobic respiration, the final electron acceptor (i.e., the one having the highest or most positive redox potential) at the end of the ETS is an oxygen molecule (O2). This is reduced to water (H2O) by the final ETS carrier complex, which includes a terminal oxidase and usually, a cytochrome. The terminal oxidase differs between bacterial types and can be used to differentiate closely related bacteria for diagnoses. For example, the gram-negative opportunist Pseudomonas aeruginosa and the gram-negative cholera-causing Vibrio cholerae use cytochrome c oxidase, which can be detected using the oxidase test, whereas other gram-negative bacteria like E. coli and other members of the Enterobacteriaceae, are negative for this test because they produce different cytochrome oxidase types.
There are many circumstances under which aerobic respiration is not possible, including any one or more of the following:
- The cell lacks genes encoding an appropriate cytochrome oxidase for transferring electrons to oxygen at the end of the electron transport system.
- The cell lacks genes encoding enzymes to minimize the severely damaging effects of dangerous oxygen radicals produced during aerobic respiration, such as hydrogen peroxide (H2O2) or superoxide (O2–).
- The cell lacks a sufficient amount of oxygen to carry out aerobic respiration.
Anaerobic Respiration
One possible alternative to aerobic respiration is anaerobic respiration, using an inorganic molecule other than oxygen as a final electron acceptor. There are many types of anaerobic respiration found in bacteria and archaea. Denitrifiers are important soil bacteria that use nitrate (NO3–) and nitrite (NO2–) as final electron acceptors, producing nitrogen gas (N2). Many aerobically respiring bacteria, including E. coli, switch to using nitrate as a final electron acceptor and producing nitrite when oxygen levels have been depleted.
Microbes using anaerobic respiration commonly have an intact Krebs cycle, so these organisms can access the energy of the NADH and FADH2 molecules formed. However, anaerobic respirers use altered ETS carriers encoded by their genomes, including distinct complexes for electron transfer to their final electron acceptors. Smaller electrochemical gradients are generated from these electron transfer systems, so less ATP is formed through anaerobic respiration. The energy yield differences are explained by the differences in . As depicted in Figure 8.19, reduction of oxygen to water is at the bottom of the redox tower while alternate terminal electron acceptors are higher up the tower.
Denitrification
Denitrification is the utilization of nitrate (NO3−) as the terminal electron acceptor. Nitrate, like oxygen, has a relatively positive reduction potential. This process is widespread, and used by many members of the Proteobacteria. Many denitrifying bacteria can also use ferric iron (Fe3+) and different organic electron acceptors. Denitrification involves the stepwise reduction of nitrate to nitrite (NO2–), nitric oxide (NO), nitrous oxide (N2O), and, eventually, to dinitrogen (N2).
Some organisms (e.g. E. coli) only produce nitrate reductase, the enzyme that catalyzes the first reduction leading to the accumulation of nitrite. Others (e.g. Paracoccus denitrificans or Pseudomonas stutzeri) reduce nitrate completely. Complete denitrification is an environmentally significant process because nitrous oxide is a powerful greenhouse gas, while nitric oxide reacts with sunlight and ozone to produce nitric acid, a component of acid rain. Denitrification is also important in biological wastewater treatment, where it can be used to reduce the amount of nitrogen released into the environment, thereby reducing eutrophication.
Denitrification takes place under special conditions in both terrestrial and marine ecosystems, where oxygen consumption exceeds the oxygen supply and sufficient quantities of nitrate are present. These environments may include certain soils and groundwater, wetlands, oil reservoirs, and in sea floor sediments.
Sulphate and Sulphur Reduction
Sulfate reduction uses sulphate (SO42-) as the electron acceptor, producing hydrogen sulphide (H2S) as a metabolic end product. Sulfate reduction is a relatively energetically poor process, though it is a vital mechanism for bacteria and archaea living in oxygen-depleted, sulphate-rich environments. Many sulphate-reducing organisms are organotrophic, using carbon compounds, such as lactate and pyruvate (among many others) as electron donors. Other sulphate reducers are lithotrophic, using hydrogen gas (H2) as an electron donor. Some species of sulphate-reducing bacteria (SRBs; e.g., Desulfovibrio sulfodismutans, Desulfocapsa thiozymogenes, and Desulfocapsa sulfoexigens) are capable of sulphur disproportionation (splitting one compound into two different compounds, in this case an electron donor and an electron acceptor) using elemental sulphur (S0), sulphite (SO32−), and thiosulphate (S2O32−) to produce both hydrogen sulphide (H2S) and sulphate (SO42−).
Sulfate-reducing bacteria are common in anaerobic environments (such as seawater, sediment, and water rich in decaying organic material) where they aid in the degradation of organic materials. In these anaerobic environments, fermenting bacteria extract energy from large organic molecules; the resulting smaller compounds (such as organic acids and alcohols) are further oxidized by acetogens, methanogens, and the competing SRBs. Acetogens and methanogens are further discussion in Methanogens and Syntrophy.
Many bacteria reduce small amounts of sulphates in order to synthesize sulphur-containing cell components; this is known as assimilatory sulphate reduction. By contrast, sulphate-reducing bacteria reduce sulphate in large amounts to obtain energy and expel the resulting sulphide as waste; this is known as “dissimilatory sulphate reduction”. Most SRBs can also reduce other oxidized inorganic sulphur compounds, such as sulphite, thiosulphate, or elemental sulphur (S0).
Toxic hydrogen sulphide is one waste product of sulphate-reducing bacteria; its rotten egg odour is often a marker for the presence of SRBs. Common examples are salt marshes and mud flats. Much of the hydrogen sulphide will react with metal ions in the water to produce metal sulphides. These metal sulphides, such as ferrous sulphide (FeS), are insoluble and often black or brown. Thus, the black colour of sludge on a pond, or in the Winogradsky sulphuretum (Figure 8.21), is due to metal sulphides that result from the action of sulphate-reducing bacteria.
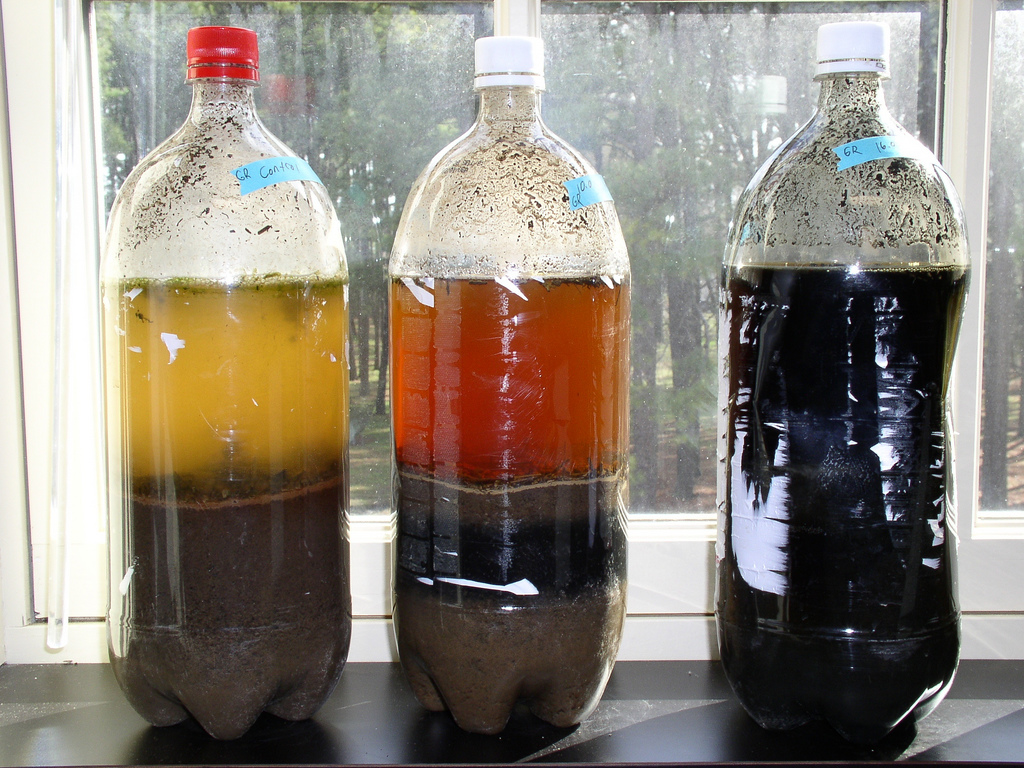
Other Alternative Electron Acceptors
Ferric iron (Fe3+) is a widespread anaerobic terminal electron acceptor used by both autotrophic and heterotrophic organisms. Electron flow in these organisms is similar to those in electron transport, ending in oxygen or nitrate, except that in ferric iron-reducing organisms the final enzyme in this system is a ferric iron reductase. Since some ferric iron-reducing bacteria (e.g. Geobacter metallireducens) can use toxic hydrocarbons (e.g. toluene) as a carbon source, there is significant interest in using these organisms as bioremediation agents in ferric iron contaminated aquifers.
Other examples of anaerobic respiration include the reduction of Manganic ion (Mn4+) to manganous (Mn2+), Selenate (SeO42−) to selenite (SeO32−) to selenium (Se), Arsenate (AsO43−) to arsenite (AsO33-), and Uranyl (UO22+) to uranium dioxide (UO2)
Organic compounds may also be used as electron acceptors in anaerobic respiration. These include the reduction of fumarate to succinate and dimethyl sulfoxide (DMSO) to dimethyl sulphide (DMS).
Methanogenesis is another form of anaerobic respiration involving the reduction of CO2. It is discussed in Methanogens and Syntrophy.
- What are the functions of the proton motive force?
- What are examples of different electron acceptors in anaerobic respiration?
Energy Yield
The number of ATP molecules generated from the ETS varies depending upon the ΔEo‘ between the initial electron donor and the terminal electron acceptor. In aerobic respiration in mitochondria, the passage of electrons from one molecule of NADH generates enough proton motive force to make three ATP molecules by oxidative phosphorylation, whereas the passage of electrons from one molecule of FADH2 generates enough proton motive force to make only two ATP molecules. Thus, the 10 NADH molecules made per glucose during glycolysis, the transition reaction, and the Krebs cycle carry enough energy to make 30 ATP molecules, whereas the two FADH2 molecules made per glucose during these processes provide enough energy to make four ATP molecules. Overall, the theoretical maximum yield of ATP made during the complete aerobic respiration of glucose is 38 molecules, with four being made by substrate-level phosphorylation and 34 being made by oxidative phosphorylation (Figure 8.22). In reality, the total ATP yield is usually less, ranging from one to 34 ATP molecules, depending on whether the cell is using aerobic respiration or anaerobic respiration and whether the source of electrons is organic or inorganic (with the exception of H2, generally much less than an organic source). In eukaryotic cells, some energy is expended to transport intermediates from the cytoplasm into the mitochondria, affecting ATP yield.
The figure below summarizes the theoretical maximum yields of ATP from various processes during the complete aerobic respiration of one glucose molecule.
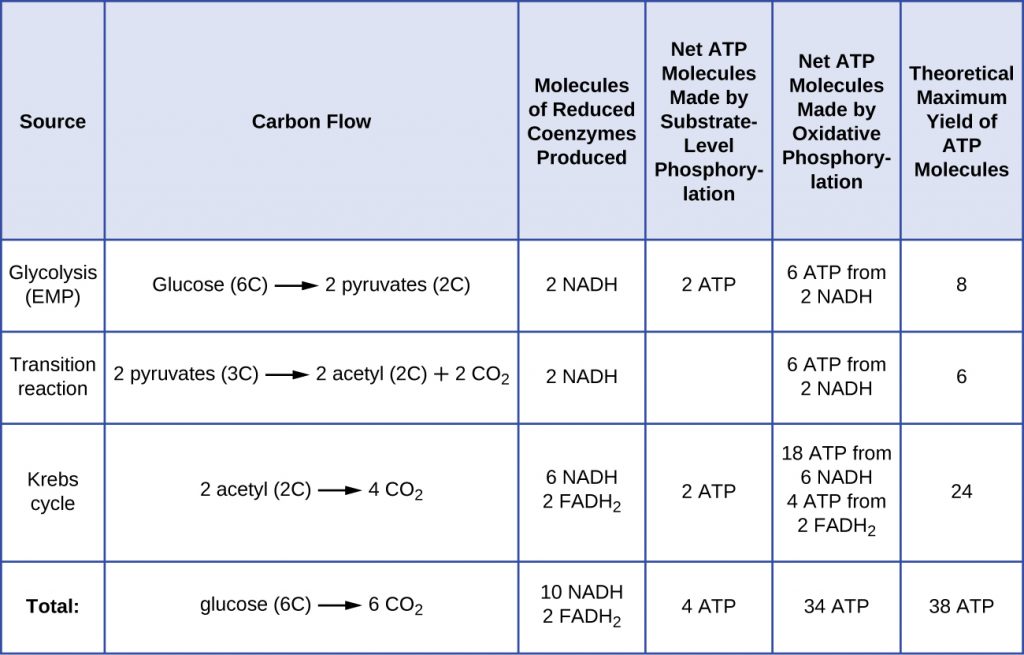
Facultative anaerobes are bacteria and archaea that are capable of aerobic respiration, as well as anaerobic metabolism, anaerobic respiration or fermentation. Given the differences in energy yields (Table 8.2), they will switch to less energetically-favourable forms of metabolism only when O2 is depleted.
Table 8.2. Comparison of energy yields between aerobic and anaerobic respiration
Comparison of energy yields from aerobic respiration versus anaerobic respiration | ||||
---|---|---|---|---|
Type of Metabolism | Example | Final Electron Acceptor | Pathways Involved in ATP Synthesis (Type of Phosphorylation) | Maximum Yield of ATP Molecules |
Aerobic respiration | Pseudomonas aeruginosa | O2 | EMP glycolysis (SLP)
Krebs cycle (SLP) Electron transport and chemiosmosis (OP): |
2
2 34 |
Total | 38 | |||
Anaerobic respiration | Paracoccus denitrificans | NO3−,SO4−2,Fe+3,CO2
other inorganics |
EMP glycolysis (SLP)
Krebs cycle (SLP) Electron transport and chemiosmosis (OP): |
2
2 1–32 |
Total | 5–36 |
- Why is anaerobic respiration less energetically favourable than aerobic respiration?
Key Takeaways
- Most ATP generated during the cellular respiration of glucose is made by oxidative phosphorylation.
- An electron transport system (ETS) is composed of a series of membrane-associated protein complexes and associated mobile accessory electron carriers. The ETS is embedded in the cytoplasmic membrane of prokaryotes and the inner mitochondrial membrane of eukaryotes.
- Each ETS complex has a different redox potential, and electrons move from electron carriers with more negative redox potential to those with more positive redox potential.
- To carry out aerobic respiration, a cell requires oxygen as the final electron acceptor. A cell also needs a complete Krebs cycle, an appropriate cytochrome oxidase, and oxygen detoxification enzymes to prevent the harmful effects of oxygen radicals produced during aerobic respiration.
- Organisms performing anaerobic respiration use alternative electron transport system carriers for the ultimate transfer of electrons to the final non-oxygen electron acceptors.
- Microbes show great variation in the composition of their electron transport systems, which can be used for diagnostic purposes to help identify certain pathogens.
- As electrons are passed from NADH and FADH2 through an ETS, the electron loses energy. This energy is stored through the pumping of H+ across the membrane, generating a proton motive force.
- The energy of this proton motive force can be harnessed by allowing hydrogen ions to diffuse back through the membrane by chemiosmosis using ATP synthase. As hydrogen ions diffuse through down their electrochemical gradient, components of ATP synthase spin, making ATP from ADP and Pi by oxidative phosphorylation.
- Aerobic respiration forms more ATP (a maximum of 34 ATP molecules) during oxidative phosphorylation than does anaerobic respiration (between one and 32 ATP molecules).
Multiple Choice
Fill in the Blank
True/False
Short Answer
- What is the relationship between chemiosmosis and the proton motive force?How does oxidative phosphorylation differ from substrate-level phosphorylation?
- How does the location of ATP synthase differ between prokaryotes and eukaryotes? Where do protons accumulate as a result of the ETS in each cell type?
Media Attributions
- Detailed redox tower
- Winogradsky Columns
- OSC_Microbio_08_03_ATPYield