10. Microbial Ecology and Applied Microbiology
10.3 Methanogens and Syntrophy
Learning Objectives
- Describe methanogenesis, the organisms that perform this type of metabolism and the environments in which it occurs
- Explain the concept of syntrophy and why it is a critical component of the anaerobic degradation of organics
- Describe how aerobic methanotrophy differs from the anaerobic oxidation of methane
- Describe the risks and benefits of biogenic methane
Methane gas is also known as natural gas. The majority of this gas, both in subsurface reserves, and in various ecosystems, is biogenic. Methanogenesis is catalyzed by a small number of related organisms in the domain Archaea, all of which have genus names with the suffix “Methano” (Figure 10.12).

Methanogens and Methanogenesis
Most methanogens are strict anaerobes. They occur in anoxic environments that are high in organics. They are microbial community members in fresh and salt-water marshes, lake and ocean sediments, hot springs, deep sea vents, animal GI tracts, and environments related to man’s activities: rice paddies, landfills and the anaerobic digesters in waste water treatment facilities.
Two common pathways of methanogenesis are the hydrogenotrophic and the acetotrophic pathways:
Hydrogenotrophic:
CO2 + 4H2 → CH4 + 2H2O
Acetotrophic:
Acetate → CH4 + CO2
Hydrogenotrophic methanogens are the more predominant type of methanogen. They are litho(auto)trophs, using hydrogen gas as their energy source and fixing CO2. Like other lithotrophs, oxidation of the H2 electron donor gives rise to a proton motive force across the plasma membrane. However, this type of methanogenesis is more complex than normal anaerobic respiration. CO2 also serves as a terminal electron acceptor, but its reduction involves a series of transfers of increasingly reduced carbon molecules between methanogen-specific cofactors. The end result is CH4 and both proton motive, and sodium motive, force. Methanogens may also derive carbon and energy from simple organic molecules such as acetic acid (acetate), formic acid (formate) and methanol; the first of these, the acetotrophic pathway, is the more common but not as well characterized as the hydrogenotrophic pathway. All of the substrates for methanogenesis, CO2, H2, and the organic molecules, are fermentation products of neighbouring organisms. These may be primary fermenters, oxidizing organic molecules NOT produced by fermentation, or secondary fermenters, which oxidize the organic end products of primary fermenters. The methanogenic degradation of organics is depicted in Figure 10.13.
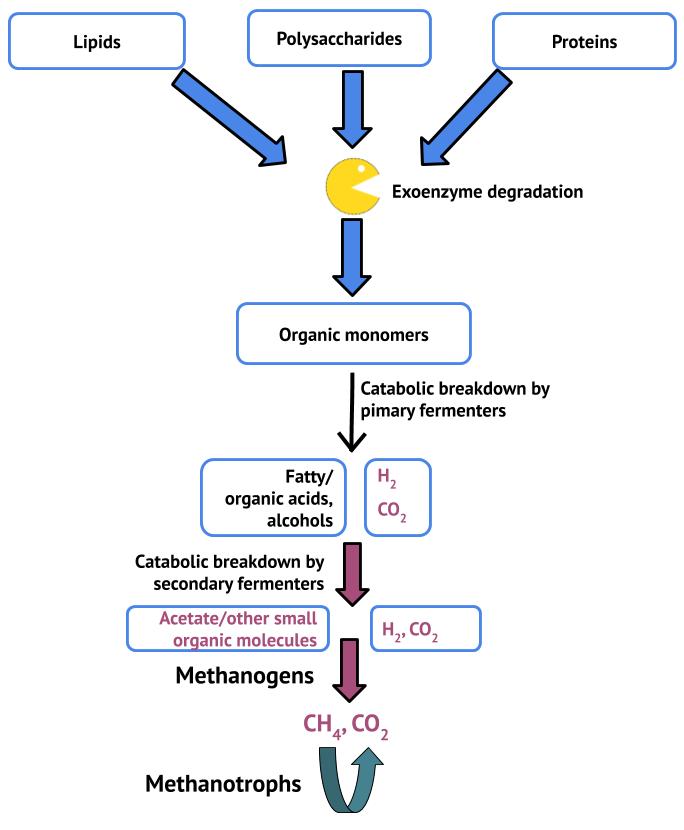
The methanogens are key players in the anaerobic carbon cycle, and combined with aerobic and anaerobic methanotrophs, which consume CH4, allow for the complete recycling of organic matter to CO2. Methanotrophy is critical, given that methane is a potent greenhouse gas with a 100 year global warming potential approximately 25 times that of CO2. In lakes and oceans, these processes are relatively well balanced, however anthropogenic methane production, i.e. production related to man’s activities, this is not the case: methanogenesis predominates.
As is true of most anaerobic metabolic processes, the energy yield from methanogenesis is not as high as from aerobic processes. For the hydrogenotrophic pathway, where the electron donor is H2 and the electron acceptor is CO2, the is positive, so the reaction yields energy. For aerobic methanotrophs, which would be exposed to methane gas bubbles percolating up through a water column, the
is also positive (Figure 10.14).
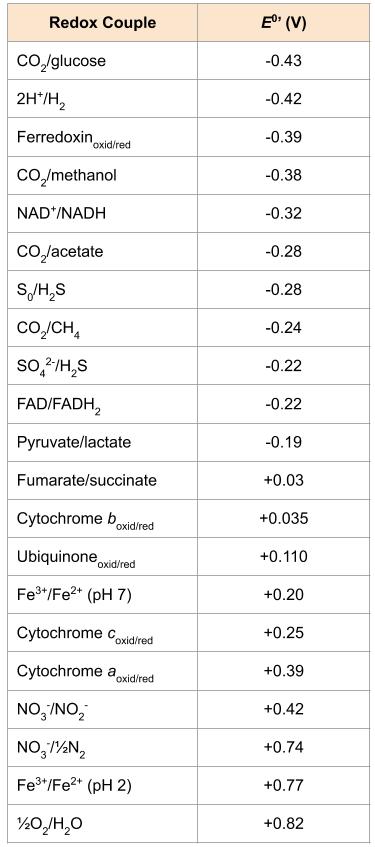
- Who are the methanogens and why are they important?
- How does the hydrogenotrophic pathway differ from the acetotrophic pathway?
- What is the relevance of the methanotrophs?
Syntrophy and Methanogenesis
Unlike heterotrophs performing aerobic or anaerobic respiration, primary fermenters gain ATP only through substrate level phosphorylation. Some of that ATP is used to generate PMF. In addition, the fermentation products are incompletely oxidized organic molecules and therefore still contain some energy. However, fermentation of glucose, using any of the myriad fermentation pathways that exist, is still exergonic:
Primary fermentations
Glucose + 4H2O → 2 acetate + 2HCO3– + 4H+ + 4H2 -207 kJ/reaction
Glucose + 2H2O → butyrate + 2HCO3– + 3H+ + 2H2 -135 kJ/reaction
Secondary fermenters, on the other hand, may be regarded as thermodynamic extremophiles. They use the organics released by the primary fermenters as their carbon and energy sources, despite the standard Gibbs free energy change of their fermentation reactions being positive:
Secondary fermentations:
Butyrate + 2H2O → 2 acetate + H+ + 2H2 +49 kJ/reaction
Benzoate + 7H2O → 3 acetate + HCO3– + 3H+ + 3H2 +70 kJ/reaction
In order to grow, secondary fermenters such as Syntrophomonas and Syntrophus, rely on a type of metabolic cooperation called syntrophy: literally, “cross feeding”. Recall from Energy, Redox Reactions and Enzymes that the is the standard free energy change of a reaction when the concentration of reactants and products is the same. For the secondary fermenters, the ability to derive energy from a substrate such as butyrate or benzoate is dependent upon their H2 end-product and methanogenic partners that rapidly consume that H2. The reduction in concentration of the H2 end product shifts the ΔG of the secondary fermentation to a negative sign, making it exergonic. In contrast, the hydrogenotrophic pathway of methanogenesis on its own, is exergonic:
HCO3– + 4 H2 + H+ → CH4 + 3H2O -130.7 kJ/reaction
The basis for most types of syntrophy is interspecies H2 transfer: H2 production by the syntroph, and H2 consumption by its partner. The most common type is that involving the methanogens. However, recently the anaerobic oxidation of methane (AOM) has been described, and this is another example of a catabolic process that on its own, should not yield energy. While not well characterized, in some cases, it appears to be mediated by a syntrophic aggregation of methanotrophic archaea and sulphate-reducing bacteria. In others, the syntrophic pairings are able to oxidize methane with nitrate instead of sulphate. Anaerobic methanotrophy is an important process in reducing the emission of methane from the ocean into the atmosphere. It is estimated that almost 90% of all the methane that arises from marine sediments is oxidized anaerobically.
- Why are syntrophs regarded as thermodynamic extremophiles?
- Describe syntrophy as it relates to secondary fermenters and the methanogens.
- What is AOM and how does it relate to syntrophy?
Biogenic Methane: Benefits and Risks
Methane absorbs infrared light and is a potent greenhouse gas, but it is also a renewable energy source. Biogenic methane can be collected and used as a sustainable alternative to fossil fuels. In fact, although older waste water treatment plants burn the CH4 from their anaerobic digesters (releasing CO2, a less powerful green-house gas), some of the newer plants trap the methane, using it to power their energy requirements.
In aquatic and marine environments, methanogenesis is largely balanced by methanotrophy. However atmospheric levels have been increasing, as have CO2 levels, since the advent of the industrial age, and while CO2 levels are roughly 200x those of CH4, methane is considerably more potent, and its atmospheric concentration has increased at almost 4x the rate of CO2. Natural wetlands are by far the major source, followed by numerous anthropogenic sources: rice agriculture, ruminants, the energy sector and waste disposal (biomass burning + landfills)[1]. In the rumen of cattle and other ruminants, anaerobic organisms, including methanogens, cooperate to digest cellulose into forms usable by the animal. Without these microorganisms, ruminants would not be able to break down the plant material that represents their entire diet. With an increasing global population has come an increase in cattle and dairy farming, and this is often accompanied by the felling of trees to create pasture. Given that an average cow emits an estimated 250 litres of methane per day (in addition to CO2), and that methane represents a loss of potential energy, methane mitigation in cattle is an active area of research. Cows with fistulas (Figure 10.15) are a common site at veterinary schools; these fistulas allow scientists to access the rumen fluid and hopefully, will one day lead to discoveries that will be of benefit to cattle, cattle farmers, and the planet.
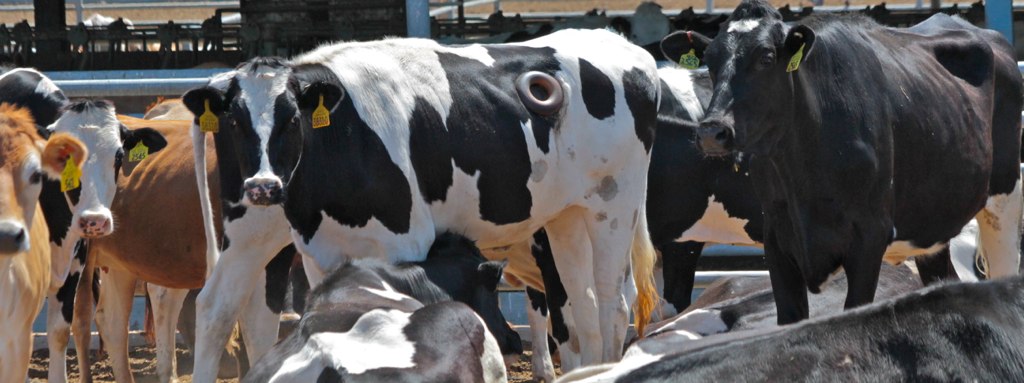
Methane Hydrates
Methane hydrates are reservoirs of methane in the form of CH4 molecules trapped within a lattice of hydrogen-bonded, frozen water molecules (Figure 10.16). They form under very specific high pressure/low temperature conditions that are associated with marine sediments around continental margins and below arctic permafrost. As long as surrounding temperatures do not rise, these hydrates are stable, and represent massive reservoirs of natural gas, conservatively estimated at ~1.8×103 Gt carbon[2]. These reservoirs are therefore of tremendous interest as a potential supply of energy. However, in the face of a warming planet, they also have implications for future climate change. Although there is considerable uncertainty about how much of the hydrates are susceptible to destabilization or “melting”, there is no question that if a tipping point were reached and there was a sudden and massive release of methane, this would also represent a tipping point for the ability of the methanotrophs to consume that methane. Recent evidence suggests that such events have happened over the past millenia, long before the advent of industrialization[3].
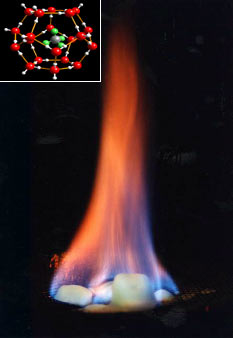
Watch this YouTube video to learn more about methane hydrates and the research being done by the US Geological Survey Gas Hydrates lab.
- What is meant by the terms biogenic methane and anthropogenic methane?
- What is the biggest source of atmospheric methane? How might that change with climate change?
- What is the concern about methane and ruminant animals?
Key Takeaways
- Biogenic methane is the primary source of ancient and current methane. It is produced by certain species of methanogenic archaea
- A common form of methanogenesis is hydrogenotrophy. These organisms are lithotrophs, using H2 as their energy source, and CO2 as both a carbon source, and terminal electron acceptor. However, the process is more complex than having a simple cytochrome oxidase that reduces CO2: oxidation of the hydrogen gives rise to proton motive, and sodium motive, force, biomass, and CH4
- Other types of methanogenesis are less well understood, but involve small organic molecules molecules such as acetate as energy sources. These species are heterotrophs
- The substrates for methanogenesis are generally the products of fermentation. Bacteria that perform secondary fermentations can be described as thermodynamic extremophiles. They form metabolic partnerships with methanogenic archaea, in order to alter the energetics of the fermentation from an endergonic reaction to an exergonic reaction. This is syntrophy.
- Syntrophy is typically an interspecies transfer of hydrogen gas. The syntrophs cannot grow without their metabolic partner consuming the H2, however the partner, typically a methanogen, does not exhibit the same dependency, since oxidation of their energy source is exergonic on its own
- In marine and aquatic environments, methanogenesis is largely balanced by methanotrophy.
- Aerobic methanotrophy is an energetically favourable reaction but the recently discovered anaerobic oxidation of methane (AOM) is not, and also requires a syntrophic partner such as sulphate-reducing bacteria.
- Methane is a major greenhouse gas. Anthropogenic methane is produced by methanogens but results from man’s activities. These include rice agriculture, cattle and dairy farming, landfills, wastewater treatment plants. Anthropogenic methane production is not balanced by methanotrophy.
- Methane hydrates are huge reservoirs of methane trapped in ice. They represent a possible source of energy, but also a hazard as they are very heat sensitive.
Multiple Choice
Drag and Drop
Short Answer
- Why would Syntrophus species be unable to grow in pure culture?
Critical Thinking
- Explain why methane hydrates pose potential risks as well as benefits.
Media Attributions
- Anaerobic degradation of organics
- Detailed redox tower
- cow with hole in side
- Burning_hydrate_inlay_US_Office_Naval_Research
- microbiology sign © Nick Youngson
- IPCC (Intergovernmental Panel on Climate Change). "Climate Change 2001: The Scientific Basis." New York, NY: Cambridge University Press, 2001. ↵
- Boswell, R. & Collett, T. S. "Current perspectives on gas hydrate resources." Energy and Environmental Science 4, 1206-1215 (2011). ↵
- Serov, P., Vadakkepuliyambatta, S., Mienert, J., Patton, H., Portnov, A., Silyakova, A., Panieri, G., Carroll, M.L., Carroll, J., Andreassen, K. and Hubbard, A. 2017. "Postglacial response of Arctic Ocean gas hydrates to climatic amelioration." PNAS 114:6215-6220. https://doi.org/10.1073/pnas.1619288114 ↵