10. Microbial Ecology and Applied Microbiology
10.6 Biogeochemical Cycles
Learning Objectives
- Define and describe the importance of microorganisms in the biogeochemical cycles of carbon, nitrogen, and sulphur
- Distinguish between the oxidation reactions and the reduction reactions in the carbon, nitrogen and sulphur cycles
- Explain why the microbes are recognized as the “biological infrastructure” of the planet
Energy flows directionally through ecosystems, entering as sunlight for phototrophs or as inorganic molecules for chemoautotrophs. The six most common elements associated with organic molecules—carbon, hydrogen, nitrogen, oxygen, phosphorus, and sulphur—take a variety of chemical forms and may exist for long periods in the atmosphere, on land, in water, or beneath earth’s surface. Geologic processes, such as erosion, water drainage, the movement of the continental plates, and weathering, all are involved in the cycling of elements on earth. Because geology and chemistry have major roles in the study of this process, the recycling of chemical matter between living organisms and their nonliving environment is called a biogeochemical cycle. Here, we will focus on the function of microorganisms in these cycles, which play roles at each step. The reactions in these cycles are all redox reactions: in one direction, a compound is oxidized, and in the reverse direction, it is reduced. Because some of those reactions are primarily, or exclusively, performed by the microbes, specifically the bacteria and archaea, the microbes are critical to the continued function of the biosphere [1] and have been described as the “biological infrastructure” of the planet.
Carbon Cycle
Carbon is one of the most important elements to living organisms, as shown by its abundance and presence in all organic molecules. The carbon cycle exemplifies the connection between organisms in various ecosystems. Carbon is exchanged between heterotrophs and autotrophs within and between ecosystems, primarily by way of atmospheric CO2, a fully oxidized version of carbon that serves as the basic building block that autotrophs use to build multi-carbon, high-energy organic molecules such as glucose (see Biosynthesis). Photoautotrophs and chemoautotrophs (i.e. the lithotrophs) harness energy from the sun and from inorganic chemical compounds, respectively, to covalently bond carbon atoms together into reduced organic compounds. The potential energy in these molecules can then be accessed through the processes of respiration and fermentation (Figure 10.32). Overall, there is a constant exchange of CO2 between the heterotrophs (which produce CO2 as a result of respiration or fermentation) and the autotrophs (which use the CO2 for fixation).
Reduced one-carbon compounds like methane accumulate in certain anaerobic environments when methanogens use CO2 as a terminal electron acceptor in a complex form of anaerobic respiration (see Methanogens and Syntrophy). These organisms are present in any anaerobic environment where there are organic molecules: in soil, wetlands and aquatic environments, but also in the guts of animals, sewage treatment plants and landfills. Aerobic and anaerobic methanotrophs are heterotrophs that consume the methane, releasing the less potent greenhouse gas, CO2.
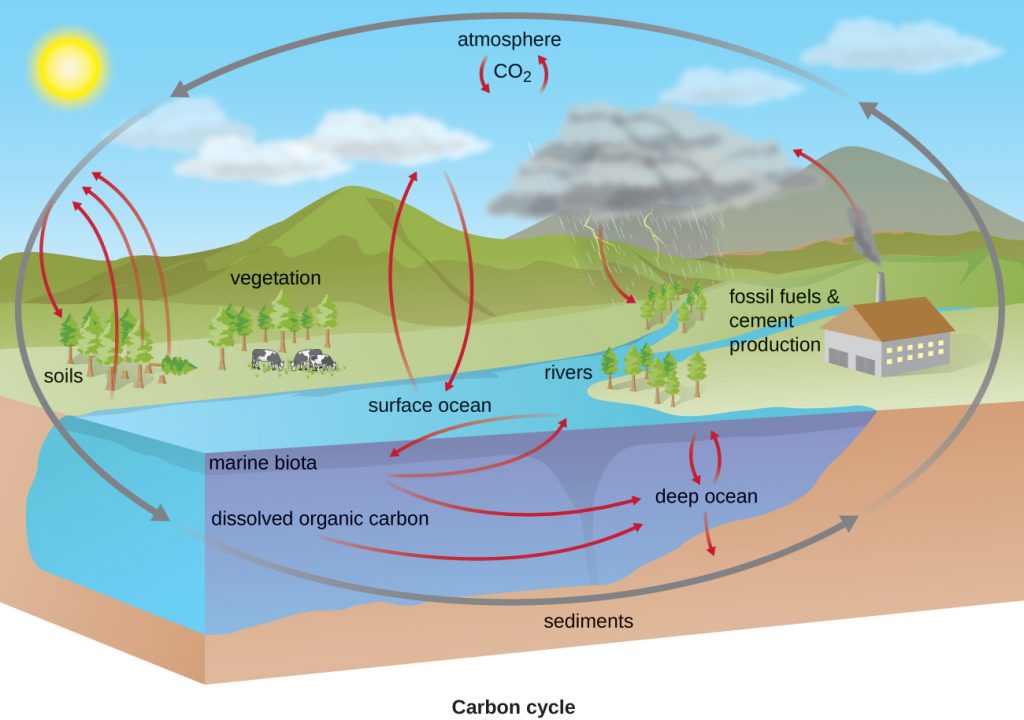
Xenobiotics
Microbes can also metabolize xenobiotics, which are synthetic organic compounds that are present in the natural environment as a direct result of pollution. Such environmental contamination may involve drugs, polychlorinated biphenyls (PCBs), trinitrotoluene (TNT), organic solvents, pesticides, plastics and products of the combustion of gasoline and oil. Many xenobiotics resist breakdown, and some accumulate in the food chain after being consumed or absorbed by fish and wildlife, which, in turn, may be eaten by humans. Of particular concern are contaminants like polycyclic aromatic hydrocarbon (PAH), a carcinogenic xenobiotic found in crude oil, and trichloroethylene (TCE), a common groundwater contaminant.
Section 10.5 discussed bioremediation of polluted water. Because of their various uses, xenobiotics are often contaminants of soil. Bioremediation processes to degrade xenobiotics to CO2 can be performed in situ or ex situ. In situ bioremediation occurs at the site of contamination. In ex situ bioremediation, contaminated material is removed and treated offsite under controlled conditions. Some bioremediation processes rely on microorganisms that are indigenous to the contaminated site or material. Through adaptation, including spontaneous mutations combined with selective pressure, with prolonged exposure, certain heterotrophic microbes acquire the ability to degrade these pollutants. Enhanced bioremediation techniques, which may be done in situ or ex situ (e.g. in bioreactors), involve the addition of nutrients and/or air to encourage the growth of the pollution-degrading microbes; they may also involve the addition of non-native microbes known to degrade contaminants. For example, certain bacteria of the genera Rhodococcus and Pseudomonas are known for their ability to fully degrade many environmental contaminants, including aromatic compounds like those found in oil, to CO2.
- What is the relationship between the heterotrophs and autotrophs?
- What is a xenobiotic and what strategies are used for environmental cleanup of xenobiotic pollution?
- Which carbon cycle reactions are exclusively performed by bacterial and archaean species?
Nitrogen Cycle
Many biological macromolecules, including proteins and nucleic acids, contain nitrogen; however, getting nitrogen into living organisms is difficult. Prokaryotes play essential roles in the nitrogen cycle (Figure 10.33), transforming nitrogen between various forms for their own needs, benefiting other organisms indirectly. Plants and phytoplankton cannot incorporate nitrogen from the atmosphere (where it exists as tightly bonded, triple covalent N2), even though this molecule composes approximately 78% of the atmosphere. Nitrogen enters the living world through free-living and symbiotic diazotrophic bacteria and archaea, which incorporate nitrogen into their macromolecules through the process of nitrogen fixation, an assimilatory reduction reaction. All diazotrophs perform this initial reduction reaction using the nitrogenase enzyme (see Nitrogen Fixation), fixing nitrogen gas (N2) into ammonia (NH3) which in turn, immediately enters biosynthetic pathways to become incorporated into biological macromolecules. Cyanobacteria are major primary producers in aquatic and marine ecosystems, but also major sources of fixed nitrogen. As discussed in Rhizobia and Biofertilization, the rhizobia fix nitrogen symbiotically in the root nodules of legumes (such as beans, peanuts, and peas). The relationship provides the plants with needed organic nitrogen while the heterotrophic bacteria receive fixed carbon as sugar in exchange. Diazotrophy was first discovered in the free-living bacterium Azotobacter, by one of the first microbial ecologists, Martinus Beijerinck, in the early 1900s (see The Birth of Microbial Ecology). This ability is found in a variety of other, unrelated, bacteria and archaea, indicating a spread through horizontal gene transfer (see How Asexual Prokaryotes Achieve Genetic Diversity). Importantly, however, it is not found in the eukaryotes.
Organic nitrogen is eventually converted back to nitrogen gas by microbes. The most well known process is through ammonification, nitrification, and finally, denitrification. The first step, ammonification, is catalyzed by certain bacteria and fungi when they convert nitrogenous waste from living animals, or from the remains of dead organisms, into ammonia (NH3). This ammonia is then oxidized to nitrite (NO2−), then to nitrate (NO3−), by aerobic nitrifying bacteria and archaea. Prominent soil nitrifiers include members of the genus Nitrosomonas and Nitrobacter, respectively. Alternatively, comammox bacteria completely oxidize ammonia to nitrate (see Nitrogen oxidizers: nitrifiers & comammox bacteria). Lastly, the process of denitrification occurs, whereby anaerobic bacteria, such as members of the genera Pseudomonas and Clostridium, use nitrate as a terminal electron acceptor in anaerobic respiration (see Anaerobic Respiration), reducing it into nitrogen gas, which then reenters the atmosphere. A similar process occurs in the marine nitrogen cycle, where these three processes are performed by marine bacteria and archaea. Recently,the process of anaerobic ammonia oxidation, or anammox, has been described. This seems to be restricted to the Planctomycete bacteria, involves the nitrite-dependent oxidation of ammonia to N2 (see Anammox), and also occurs in marine, aquatic and soil ecosystems.
As described previously, human activity releases nitrogen into the environment in the form of artificial fertilizers, which are then washed into lakes, rivers, and streams by surface runoff. A major effect from this runoff is eutrophication of aquatic and marine coastal ecosystems (see Water Pollution and Bioremediation). Eutrophication, where normally limiting nutrients become plentiful, causes a population bloom of the phytoplankton, followed by a bloom of the aerobic, heterotrophic bacteria. The final result is increased biological oxygen demand and, if the situation persists, hypoxia. The loss of dissolved oxygen leads to the death of aquatic organisms.
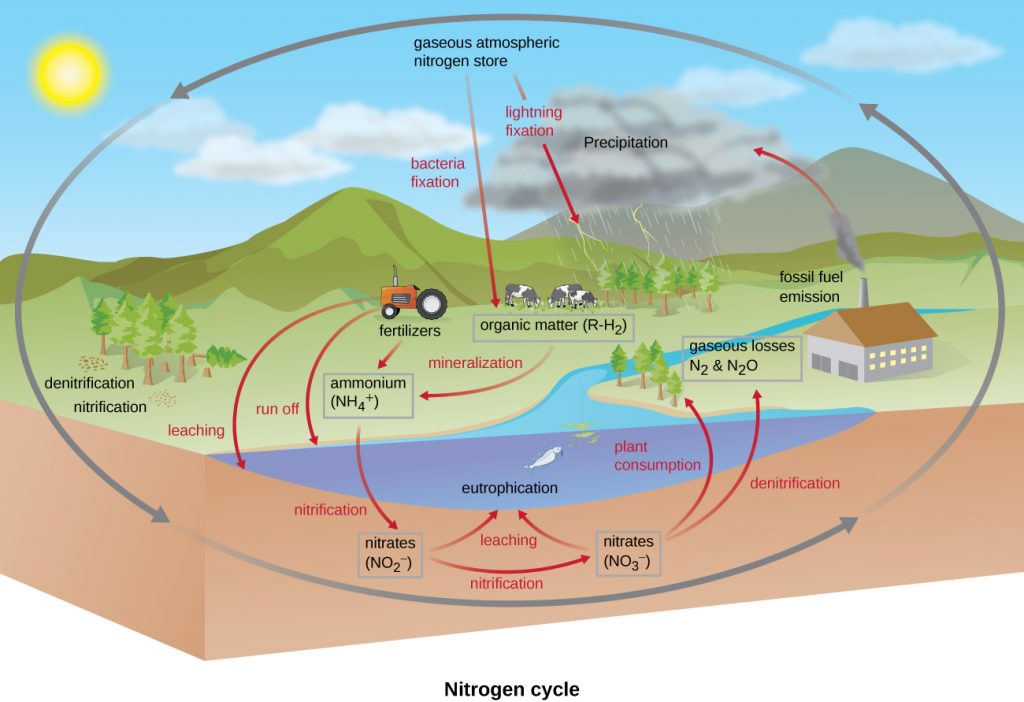
EYE ON ETHICS: Feeding the World…and the World’s Algae
Artificial fertilizers have become an important tool in food production around the world. They are responsible for many of the gains of the so-called green revolution of the 20th century, which has allowed the planet to feed many of its more than 7 billion people. Artificial fertilizers provide nitrogen and phosphorus, key limiting nutrients, to crop plants, removing the normal barriers that would otherwise limit the rate of growth. Thus, fertilized crops grow much faster, and farms that use fertilizer produce higher crop yields.
Unfortunately, careless use and overuse of artificial fertilizers have been demonstrated to have significant negative impacts on aquatic ecosystems, both freshwater and marine. Fertilizers that are applied at inappropriate times or in too-large quantities allow nitrogen and phosphorus compounds to escape use by crop plants and enter drainage systems. Inappropriate use of fertilizers in residential settings can also contribute to nutrient loads, which find their way to lakes and coastal marine ecosystems. As water warms and nutrients are plentiful, microscopic algae bloom, often changing the colour of the water because of the high cell density.
As described in Section 10. 5, algal blooms may be directly or indirectly harmful to humans or wildlife. As the algal population expands and then dies, it provides a large increase in organic matter for the aerobic heterotrophic bacteria, which also bloom, consuming the available oxygen and creating hypoxic or “dead zones” where animal life has virtually disappeared. More direct harm results from toxin-producing algae or cyanobacteria. Recurring cyanobacterial algal blooms in Lake Erie (Figure 10.31) have forced municipalities to issue drinking water bans for days at a time because of unacceptable toxin levels.
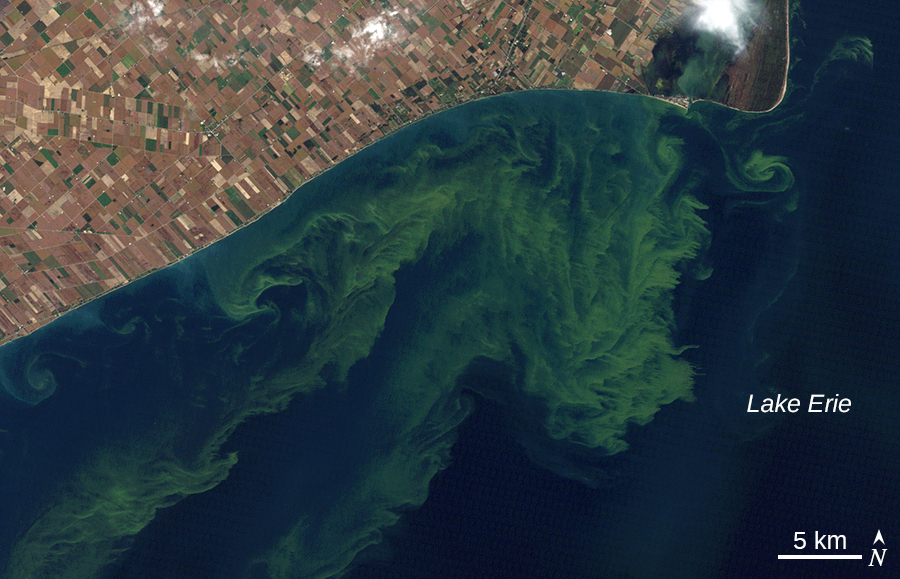
The benefits of crop fertilizer—the main cause of such blooms—are difficult to dispute. There is no easy solution to this dilemma, as a ban on fertilizers for agriculture is neither politically nor economically feasible. Some municipalities have established by-laws banning residential and green space fertilization. In the case of the Lake Erie watershed, the Province of Ontario partnered with the Government of Canada and Ducks Unlimited Canada to restore or create, a total of 92 wetlands, in part, to intercept and absorb the the runoff from surrounding farms.[2]
- Which steps in the nitrogen cycle are reductions and which are oxidations?
- Which nitrogen cycle reactions are performed exclusively by bacteria and archaea?
Sulfur Cycle
Sulfur is an essential element for the macromolecules of living organisms. As part of the amino acids cysteine and methionine, it is involved in the formation of proteins. It is also found in several vitamins necessary for the synthesis of important biological molecules like coenzyme A. Several groups of microbes are responsible for carrying out processes involved in the sulphur cycle (Figure 10.34). The anoxygenic photosynthetic bacteria (the “purple sulphurs” and the “green sulphurs” – see Microbial Phototrophy), as well as lithotrophic archaea and bacteria (Lithotrophy), use hydrogen sulphide as an electron donor, oxidizing it first to elemental sulphur (S0), then to sulphate (SO42−). This leads to stratification of hydrogen sulphide in soil, with levels increasing at deeper, more anaerobic depths.
Many bacterial and archaeal species use sulphate as a terminal electron acceptor in anaerobic respiration; this is dissimilatory reduction. In contrast, sulphate is also used by microbes, and by plants, as a sulphur source, reducing the sulphate in the process of various sulphur-assimilating biosynthetic reactions. These are therefore assimilatory reductions. Decomposition of dead organisms by fungi and bacteria remove sulphur groups from amino acids (and other S-containing organics), producing hydrogen sulphide, returning inorganic sulphur to the environment.
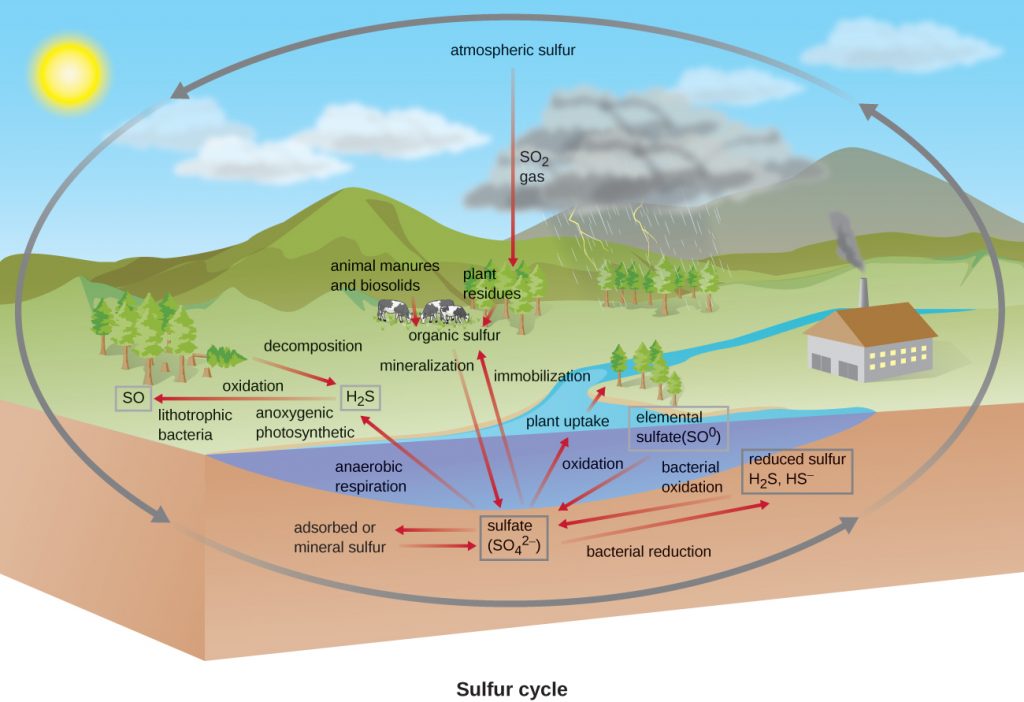
- Which groups of microbes carry out the sulphur cycle?
- What is the difference between an assimilatory and a dissimilatory reduction reaction?
Other Biogeochemical Cycles
Beyond their involvement in the carbon, nitrogen, and sulphur cycles, prokaryotes are involved in other biogeochemical cycles as well. Some of these are also based on redox reactions. In the examples of iron (Fe), manganese (Mn), and chromium (Cr) cycles, lithographic bacteria and archaea oxidize inorganic compounds, while anaerobic bacteria and archaea use the oxidized products as terminal electron acceptors, regenerating the reduced form. Several other elements undergo chemical cycles that do not involve redox chemistry. Examples of these are phosphorus (P), calcium (Ca), and silica (Si) cycles. The cycling of these elements is particularly important in oceans because large quantities of these elements are incorporated into the exoskeletons of marine organisms. These biogeochemical cycles are not based exclusively on redox chemistry; fluctuations in the solubility of compounds containing calcium, phosphorous, and silica are also critical aspects. The overgrowth of naturally occurring microbial communities is typically limited by the availability of nitrogen (as previously mentioned), phosphorus, and iron. Human activities introducing excessive amounts of iron, nitrogen, or phosphorus (typically from detergents) may lead to eutrophication.
Key Takeaways
- The recycling of inorganic matter between living organisms and their nonliving environment is called a biogeochemical cycle. Microbes play significant roles in these cycles.
- In the carbon cycle, heterotrophs degrade reduced organic molecule to produce carbon dioxide, whereas autotrophs fix carbon dioxide to produce organics. Methanogens typically form methane by using CO2 as a final electron acceptor during anaerobic respiration; methanotrophs oxidize the methane, using it as their carbon source.
- In the nitrogen cycle, nitrogen-fixing bacteria and archaea (the diazotrophs) convert atmospheric nitrogen into ammonia which is immediately incorporated into cellular biosynthetic reactions. In the reverse reaction, ammonification, N-containing waste is oxidized by heterotrophs, releasing ammonia. The ammonia can then be oxidized to nitrite and nitrate (nitrification) by lithotrophic bacteria and archaea. Nitrates are a bioavailable source of biosynthetic nitrogen for various life forms, including plants. Finally, anaerobic bacteria and archaea convert nitrate back to nitrogen gas through anaerobic respiration, or denitrification. Nitrate is therefore reduced, either in assimilatory reactions, or dissimilatory reactions.
- In sulphur cycling, some of the anoxygenic phototrophic bacteria, as well as certain lithotrophic bacteria and archaea, use hydrogen sulphide as an electron donor, producing elemental sulphur, then sulphate. Like nitrate, sulphate is a bioavailable sulphur source and can be reduced through certain biosynthetic (assimilatory) reactions. Alternatively, sulphate-reducing bacteria and archaea can use sulphate as a final electron acceptor in anaerobic respiration, a dissimilatory reaction, converting it back to hydrogen sulphide.
- Human activities that introduce excessive amounts of naturally limited nutrients (like iron, nitrogen, or phosphorus) to aquatic systems may lead to eutrophication.
- Xenobiotics are synthetic organic compounds that are often environmental pollutants. Microbial bioremediation of xenobiotics may involve the use of native, or non-native microbes to degrade the contaminants, and may be done in situ or ex situ in bioreactors. In some instances, the microbes have been engineered to degrade the pollutant, however more often, microbes have evolved the ability to degrade the xenobiotic as a result of prolonged exposure to a pollutant.
Multiple Choice
Fill in the Blank
True/False
Short Answer
- Which N-cycle reactions are associated with human activity and eutrophication?
- Identify the reactions in the N, S and C-cycles that are associated with photosynthetic bacteria.
Critical Thinking
- Compare dissimilatory reductions with assimilatory reductions, and give an example of each.
- Explain why the microbes are considered to be the biological infrastructure of the planet.
Media Attributions
- OSC_Microbio_08_07_CCycle
- OSC_Microbio_08_07_NCycle
- microbiology sign © Nick Youngson
- OSC_Microbio_08_07_SCycle
- Gilbert JA, Neufeld JD (2014) Life in a World without Microbes. PLoS Biol 12(12): e1002020. https://doi.org/10.1371/journal.pbio.1002020 ↵
- Ontario Ministry of Natural Resources. News Release, April 26, 2018. https://news.ontario.ca/mnr/en/2018/04/ontario-and-canada-support-better-water-quality-for-lake-erie.html ↵