31 CRISPR Therapeutics – Novel Use of CRISPR/Cas9 Technique in Disease Therapy
Viola Halder
CRISPR Therapeutics – Novel Use of CRISPR/Cas9 Technique in Disease Therapy
A Case Study
Created by Viola Halder
Since the advent of CRISPR/Cas9 based genome-editing technology, researchers have been quite excited about its potential applications including gene deletion, gene regulation, mutated gene repair for chronic disease therapy and much more (Menn, 2017; Tycko, Hess, Jeng, Dubreuil, & Bassik, 2017). On a slightly chilly morning – October 28, 2013 –Emmanuelle Charpentier, Rodger Novak and Shaun Foy decided to do something about this. They founded CRISPR Therapeutics (CRISPR Tx) with the mission to find and develop transformative gene-based medicines for serious diseases using CRISPR/Cas9 gene editing platform fill the void that existed in CRISPR based therapeutics (CRISPR Therapeutics, 2018a). Emmanuelle Charpentier stated in a recent interview with Alyson Weidmann the reason behind CRISPR Therapeutics (Weidmann, 2018):
I am very excited about the potential of CRISPR-Cas9 for the treatment of serious genetic diseases for which there is currently no cure but also for cancer or infectious diseases. This is one of the reasons I co-founded CRISPR Therapeutics.
The Company
CRISPR Tx started with its headquarters in Zug, Switzerland, where all of their efforts were put into research and development activities in determining CRISPR based therapeutic strategies (SEC, 2016). Initial market development was accomplished, substantial capital was raised and, in 2014, foundational intellectual property, for the use of CRISPR in human therapeutics, underlying the research was licensed to CRISPR Tx and its subsidiaries (SEC, 2016). CRISPR Tx formed CRISPR Therapeutics Limited in the United Kingdom on February of 2014 for select business operations, and CRISPR Therapeutics Incorporated in Cambridge, Massachusetts, USA for research and development operations, both wholly-owned subsidiaries (CRISPR Therapeutics, 2018a; SEC, 2016).
The goals of CRISPR Tx were outlined (see Figure 1) and included the following (CRISPR Therapeutics, 2017b):
- Focus on the hematopoietic system (related to blood) through ex vivo (within cells, but outside of the body) approaches
- Pursue select therapies requiring in vivo (within cells, inside the body) approaches
- Foster relationships with Bayer and Vertex (using joint ventures and collaborations)
- Advance our position to become leaders in the field of gene editing
As of June 2016, CRISPR Tx has raised almost $140 million, with $38 million in B-series funding (CRISPR Therapeutics, 2017a). Part of the B-series funding was from pharmaceutical firms such as GlaxoSmithKline, Vertex Pharmaceuticals and Bayer and biotech companies such as Celgene and New Enterprise Associates (CRISPR Therapeutics, 2017b). See Figure 2 for visual representation. In October 2016, after their initial public offering, the company was able to raise an additional $56 million (Gallo, Jr, Sarata, & Cowan, 2018). The company also participates in joint ventures and collaborations with a variety of different companies to find cures for diseases such as Cystic Fibrosis, Sickle cell disease, severe combined immunodeficiency and haemophilia (CRISPR Therapeutics, 2017b). CRISPR Tx also partnered up for a joint venture with Bayer AG to create a new company – Casebia Therapeutics – whose sole purpose was “to discover, develop and commercialize new breakthrough therapeutics to cure blood disorders, blindness, and congenital heart disease” (CRISPR Therapeutics, 2015). Part of the agreement included that Bayer would provide $300 million in research and development investments over the next five years for the joint venture while taking a stake in CRISPR Therapeutics for $35 million (CRISPR Therapeutics, 2015). As of March 23, 2018, CRISPR Tx’s market capitalization was $2.049 million (Bloomberg®, 2018).
The team at CRISPR Tx consisted of experts in not only CRISPR/Cas9 technology, but also in gene editing, stem cell biology, advanced drug delivery technologies, RNA interference and gene silencing (CRISPR Therapeutics, 2018a). Scientific Advisors and Investors included Stephen Elledge (renowned expert in DNA repair and damage responses), Craig Mello (Nobel Laureate in the discovery of RNAi – form of gene editing) Matthew Porteus (renowned expert in gene edition) and Dan Anderson (famous for early works in CRISPR in vivo delivery) (CRISPR Therapeutics, 2017b). With these and many more experts in the field, CRISPR Tx was well on its way to create CRISPR based therapeutics for serious human diseases. Charpentier stated that (Weidmann, 2018):
It is our aim to better understand … mechanisms and generate new findings in basic science that can possibly be translated into new biotechnological and biomedical applications, e.g., genome editing tools or anti-infective strategies.
The Technology
Targeted mutations in the mammalian genome have been the focus of genetic research since the 1980s, creating the field of genome editing (Dow, 2015). The methods related to genome editing has been the recent focus for scientists, regardless of the fact that the field is not novel (Dow, 2015). Scientists have discovered techniques that have become efficient, specific and versatile over the years (CRISPR Therapeutics, 2017b). The primary goals were to discover a technique which could allow for site-specific mutagenesis in eukaryotes (comprised on animals, plants and fungi) (Jiang, Bikard, Cox, Zhang, & Marraffini, 2013) while also providing genome editing strategies in other organisms (Gilbert et al., 2013).
A few techniques that have been the forefront of genome editing, prior to CRISPR/Cas9 system, were zinc finger nucleases (ZFNs) and TAL effector nucleases (TALENs) (Gaj, Gersbach, & Barbas III, 2013). Both had the capacity to modify genes with the induction of double-strand breaks in DNA (cutting both strands of the DNA within a cell) (Gaj et al., 2013). Once the DNA is cut, DNA repair mechanisms within the cell where damage has incurred are recruited to repair the specific genomic locations (Dow, 2015; Gaj et al., 2013). Regardless of the potential associated with ZFNs and TALENS, there are some limitations to the technologies that have frustrated scientists (Dow, 2015). For both techniques, the process required to engineer the molecules associated are quite difficult and require long and expensive steps (Dow, 2015). Aside from that, there is an intellectual property wall that exists with regards to ZFNs, that makes it challenging for scientists to apply it easily (Dow, 2015). CRISPR/Cas9 does not have these drawbacks and can be used with relative ease and accuracy.
CRISPR/Cas9 construct was discovered to be the immune system used by bacteria and other prokaryotic organisms to fight against foreign invaders (Cong et al., 2013). The system uses an RNA-dependent system (guide RNA) for sequence-specific detection and silencing of invading nucleic acids (Jinek et al., 2012). The CRISPR/Cas9 system add to the CRISPR array as the host prokaryote comes across foreign DNA (Jinek et al., 2012). The CRISPR array is formed into a CRISPR RNA (crRNA); The crRNA guides the Cas9 protein to a particular location on the DNA to allow Cas9’s endonuclease activity cleave (cut) both strands of DNA, causing a double-strand break (DSB) (Cong et al., 2013). After the DNA is cut, the cell depends on the DNA damage repair mechanisms of the host to repair the damage and return the cell to normal function (Cong et al., 2013). Cong and his colleagues (2013) created the most efficient version of CRISPR/Cas9 system (CRISPR 2.0) by discovering the important components of the original to work in mammalian (humans, mice, etc.) cells. In this version, the construct includes the Cas9 protein and a small guide RNA (sgRNA) is used to replace the crRNA (Cong et al., 2013).
While the biological definition is difficult to understand at the basic level, another way to define CRISPR/Cas9 is basically as a set of molecular scissors that can cut DNA. Then, the cell’s own repair machinery can repair the cut which results in disruption or deletion a disease-causing gene or correction that gene if the desired DNA is added as a template (CRISPR Therapeutics, 2018a). A second analogy can compare CRISPR/Cas9 technique to two keyboard shortcuts used sequentially: CTRL-F, followed by the delete key. The sgRNA functions as CTRL-F to find the target DNA of interest, and the Cas9 protein functions as the delete key to delete the DNA of interest (Menn, 2017).
Furthermore, the patent war that has existed between the Broad Institute and University of California, Berkley (UC Berkley) since 2016 (Hamermesh & Preble, 2016) has officially been resolved as of January 2018 (Servick, 2018). The Broad Institute won the patent rights in the United States but are still on the battlefield in the European landscape; they have planned to file an appeal to overturn the revocation decision (Servick, 2018). That being said, Emmanuelle Charpentier, along with Dr Ines Fonfara and the University of Vienna entered into a patent assignment agreement in November 2014 which was under investigation for interference (SEC, 2016). However, since the European Patent Office (EPO) revoked the Broad Institute’s claim for the patent, it is assumed that the EPO intends to grant the patent to UC Berkley and its affiliates (such as CRISPR Tx) (Mukherjee, 2017).
The patent would cover uses in multiple cell types, including humans – the main focus of companies developing treatments for everything from cancer to HIV/AIDS and various genetic disorders using CRISPR.
See Figure 3 for more details.
This technique has the benefit of being efficient, specific and versatile (CRISPR Therapeutics, 2017b) as outlined in Figure 4. Therefore, CRISPR/Cas9 has the implication that genes – which serve as the blueprint of all life – are not predetermined; they can be controlled, changed and moulded when needed.
The Problems and Solutions
While CRISPR/Cas9 technology has existed since its discovery in the early 2000s (Jinek et al., 2012), two problems existed. One, the technique still had to be used in humans; there needed to be a way to allow for the CRISPR/Cas9 construct to enter the cells that required genome editing without harming the rest of the body. This was made clear by Dr Feng Zhang, one of the scientists who discovered the non-prokaryotic uses of CRISPR (Weidmann, 2018):
One of the persistent challenges facing the field of genome editing is the need to be able to deliver systems with all the elements needed to efficiently and precisely integrate a DNA template into the intended location of the genome.
George Church, the principal investigator in a lab responsible for establishing CRISPR platforms for engineered organs, agreed with Dr Zhang in stating (Weidmann, 2018):
It would be nice to have higher efficiency delivery in vivo [inside the body] …
A few solutions were proposed by CRISPR Tx in order to solve this dilemma. First, an ex vivo strategy was explored in order to allow for CRISPR/Cas9 based therapeutics to succeed (CRISPR Therapeutics, 2018b). Figure 5 depicts what may happen in such a strategy: damaged cells harvested from the body (autologous or self-donated), then CRISPR is applied to them – the single gene is edited as required, and finally the edited cells are transplanted back into the patient where they can function normally (CRISPR Therapeutics, 2018a).
This problem was further explored by Dan Anderson, made famous for his early works in CRISPR in vivo delivery (CRISPR Therapeutics, 2017b; Yin et al., 2016). It was discovered, by him and others working within the company, that viral and non-viral means of CRISPR/Cas9 construct delivery into the cells can be achieved with variable results (CRISPR Therapeutics, 2018b; Yin et al., 2016). In this situation, as seen in Figure 5, the CRISPR/Cas9 construct is packaged inside a delivery vehicle of some sort and this vehicle is delivered into the body – either to the organ of interested or into the blood so it can travel systemically throughout the body (CRISPR Therapeutics, 2018a). Figure 6 outlines the different non-viral and viral delivery vehicles that were identified for use in CRISPR based therapy. These were established using various joint venture and collaborations with MIT, CureVac and StrideBio (CRISPR Therapeutics, 2017c, 2017d, 2017e).
Both in vivo and ex vivo strategies require further research to determine which will result in greater success. Research is also required for the different in vivo delivery vehicles to determine which offers the greatest benefit while reducing any unwanted toxic effects.
The second problem was to determine what type of diseases would be ideal for discovery and development of CRISPR based therapeutics? There are over 10,000 human diseases that are monogenic – a single gene in the human DNA that is mutated, causing 1 in every 100 babies to be born with the disease (Genomic Resource Centre, 2018). Tay Sachs, Cystic Fibrosis, Huntington’s disease, Sickle Cell disease (SCD) and Thalassemia are a few examples of such diseases (Genomic Resource Centre, 2018). Monogenic diseases have been at the forefront of clinical and scientific research. Scientists were curious to research whether CRISPR/Cas9 can be used to address genetic defects to determine if genetic editing is an option or not (CRISPR Therapeutics, 2018a). CRISPR Tx established some in vivo therapies based on research for Sickle Cell disease (SCD) and b-thalassemia.
There are over 300000 babies born with SCD and over 60000 babies born with b-thalassemia (Genomic Resource Centre, 2018). Both diseases are hemoglobinopathies because they cause mutation with the globin subunit (part) of haemoglobin, part of the red blood that carries oxygen and is quite important (Genomic Resource Centre, 2018). Both diseases are monogenic – a gene in chromosome 11 is mutated (changed) for both – which causes blood to be inefficient in transporting oxygen where required (Genomic Resource Centre, 2018). Both diseases are associated with anaemia (low red blood cell volume resulting in pale skin and exhaustion), pain and early death (CRISPR Therapeutics, 2018a). One approach to reducing symptoms is to go through a blood transfusion to replace the damaged cells, which increase the number of hospital stays as well as expensive (CRISPR Therapeutics, 2018a).
CRISPR Tx created an ex vivo CRISPR/Cas9 based therapy – denoted CTX001 – utilizing the fact that fetal haemoglobin has shown positive results in dealing with hemoglobinopathies.Increase in the levels of foetal haemoglobin in the blood has been known to alleviate morbidity and mortality rates for both SCD and β-thalassemia while decreasing transfusion requirements (Musallam et al., 2012; Powars, Weiss, Chan, & Schroeder, 1984).
CTX001 is an investigational CRISPR gene-edited autologous hematopoietic stem cell therapy, in which a patient’s hematopoietic stem cells are engineered to produce high levels of foetal haemoglobin in red blood cells to alleviate transfusion requirements for β-thalassemia patients and painful and debilitating sickle crises for sickle cell patients. – Emmanuelle Charpentier (Weidmann, 2018).
This novel CRISPR based therapy is currently in clinical trials in Europe for β-thalassemia and USA for SCD.
Current Pipeline/The Future
Currently, CRISPR Tx has eleven potential CRISPR based therapies in their pipeline at various stages of research (See Figure 7) (CRISPR Therapeutics, 2018b). They are either in vivo or ex vivo, and range from being causing DNA disruptions to correct mutated genes by adding the appropriate repair template (CRISPR Therapeutics, 2018a). The potential therapies also range from being wholly owned by CRISPR Tx, to being in collaboration or a joint venture with other therapy based companies such as Vertex or Casebia (CRISPR Therapeutics, 2018a). The 4 major areas for CRISPR based therapies include (i) ex vivo programs of hematopoietic cells, (ii) ex vivo programs for immuno-oncology (using the built-in immune system to fight cancer cells), (iii) in vivo programs targeting the liver and (iv) in vivo programs targeting muscle and lung systems (CRISPR Therapeutics, 2018a). Conclusion
CRISPR Therapeutics took advantage of a void that exists in the field of genomic research by bridging the gap between scientific research and the biomedical community. Emmanuelle Charpentier knew that she and her colleagues had to take advantage of the potential associated with CRISPR/Cas9 technology. She was aware that the potential applications including gene deletion, gene regulation, mutated gene repair for chronic disease therapy and much more were quite exciting and a challenge that she and all her colleagues were more than willing to take up.
When asked about the future of CRISPR technologies, Emmanuelle Charpentier was quite excited about its prospects (Weidmann, 2018):
The CRISPR-Cas technology is extremely versatile. For this reason, it was adapted around the world where scientists are applying the technology for the basic understanding of mechanisms of life in a large diversity of cells and organisms and are working on potential future applications.
I am very excited about the potential of CRISPR-Cas9 for the treatment of serious genetic diseases for which there is currently no cure but also for cancer or infectious diseases. This is one of the reasons I co-founded CRISPR Therapeutics.
References
Bloomberg®. (2018, March 23). CRISPR Therapeutics AG (Biotech). – CRSP US Equity. Retrieved March 23, 2018, from Bloomberg database
Cong, L., Ran, F. A., Cox, D., Lin, S., Barretto, R., Habib, N., … Marraffini, L. (2013). Multiplex genome engineering using CRISPR/Cas systems. Science, 39, 819–823.
CRISPR Therapeutics. (2015, December 21). Bayer and CRISPR Therapeutics AG join Forces to Discover, Develop and Commercialize Potential Cures for Serious Genetic Diseases. News and Events – Press Release. Retrieved from http://ir.crisprtx.com/phoenix.zhtml?c=254376&p=irol-newsArticle_Print&ID=2184476
CRISPR Therapeutics. (2017a). CRISPR Therapeutics Raises Additional $38M as Part of Series B Financing. News and Events – Press Release. Retrieved from http://crisprtx.com/news-events/news-events-press-releases-2016-06-24.php
CRISPR Therapeutics. (2017b, February). CRISPR Therapeutics Corporate Overview. CRISPR Therapeutics.
CRISPR Therapeutics. (2017c, April 17). CRISPR Therapeutics and Casebia Therapeutics Announce Exclusive Development and Option Agreement with StrideBio. News and Events – News Release. Retrieved from http://ir.crisprtx.com/phoenix.zhtml?c=254376&p=irol-newsArticle&ID=2262218
CRISPR Therapeutics. (2017d, May 8). CRISPR Therapeutics Announces Exclusive License of Lipid Nanoparticle Technologies Developed at MIT. News and Events – News Release. Retrieved from http://ir.crisprtx.com/phoenix.zhtml?c=254376&p=irol-newsArticle&ID=2270759
CRISPR Therapeutics. (2017e, November 13). Collaborate with CureVac on mRNA for Gene-Editing Programs. News and Events – News Release. Retrieved from http://ir.crisprtx.com/phoenix.zhtml?c=254376&p=irol-newsArticle&ID=2316361
CRISPR Therapeutics. (2018a). CRISPR Therapeutics AG. Retrieved April 7, 2018, from http://crisprtx.com/index.php
CRISPR Therapeutics. (2018b, March). CRISPR Therapeutics Corporate Overview. CRISPR Therapeutics.
Dow, L. E. (2015). Modeling disease in vivo with CRISPR/Cas9. Trends in Molecular Medicine, 21(10), 609–621.
Gaj, T., Gersbach, C. A., & Barbas III, C. F. (2013). ZFN, TALEN, and CRISPR/Cas-based methods for genome engineering. Trends in Biotechnology, 31(7), 397–405.
Gallo, M. E., Jr, J. F. S., Sarata, A. K., & Cowan, T. (2018). Advanced Gene Editing: CRISPR-Cas9. Congressional Research Service, 38.
Genomic Resource Centre. (2018). WHO | Genes and human disease. Retrieved April 5, 2018, from http://www.who.int/genomics/public/geneticdiseases/en/index2.html#Thalassaemia
Gilbert, L. A., Larson, M. H., Morsut, L., Liu, Z., Brar, G. A., Torres, S. E., … Doudna, J. A. (2013). CRISPR-mediated modular RNA-guided regulation of transcription in eukaryotes. Cell, 154(2), 442–451.
Hamermesh, R. G., & Preble, M. G. (2016). The CRISPR-Cas9 Quarrel. Retrieved from https://www.hbs.edu/faculty/Pages/item.aspx?num=51816
Jiang, W., Bikard, D., Cox, D., Zhang, F., & Marraffini, L. A. (2013). RNA-guided editing of bacterial genomes using CRISPR-Cas systems. Nature Biotechnology, 31(3), 233.
Jinek, M., Chylinski, K., Fonfara, I., Hauer, M., Doudna, J. A., & Charpentier, E. (2012). A programmable dual-RNA–guided DNA endonuclease in adaptive bacterial immunity. Science, 337, 816–821.
Menn, A. (2017, December 2). German biotechs embrace CRISPR gene-editing technology. Handelsblatt Global Edition. Retrieved from https://global.handelsblatt.com/companies/german-biotechs-embrace-crispr-gene-editing-technology-856539
Mukherjee, S. (2017, March 28). The Patent Battle Over Revolutionary CRISPR Gene-Editing Tech Just Went Global. Retrieved April 8, 2018, from http://fortune.com/2017/03/28/crispr-patent-crispr-therapeutics/
Musallam, K. M., Sankaran, V. G., Cappellini, M. D., Duca, L., Nathan, D. G., & Taher, A. T. (2012). Fetal hemoglobin levels and morbidity in untransfused patients with β-thalassemia intermedia. Blood, 119(2), 364–367.
Powars, D. R., Weiss, J. N., Chan, L. S., & Schroeder, W. A. (1984). Is there a threshold level of fetal hemoglobin that ameliorates morbidity in sickle cell anemia? Blood, 63(4), 921–926.
SEC. (2016). SEC Info – CRISPR Therapeutics AG – ‘10-Q’ for 6/30/17. United States Securities and Exchange Commission, 6–30, 54.
Servick, K. (2018, January 24). European Patent Office Revokes Broad Institutes Patent – CRISPR Update. Retrieved April 8, 2018, from http://www.crisprupdate.com/european-patent-office-revokes-broad-institutes-patent/
Tycko, J., Hess, G. T., Jeng, E. E., Dubreuil, M., & Bassik, M. C. (2017). The expanding CRISPR toolbox. Nature.
Weidmann, A. G. (2018). Frontiers in CRISPR. ACS Chemical Biology, 13, 296–304.
Yin, H., Song, C.-Q., Dorkin, J. R., Zhu, L. J., Li, Y., Wu, Q., … Bizhanova, A. (2016). Therapeutic genome editing by combined viral and non-viral delivery of CRISPR system components in vivo. Nature Biotechnology, 328.
Figures
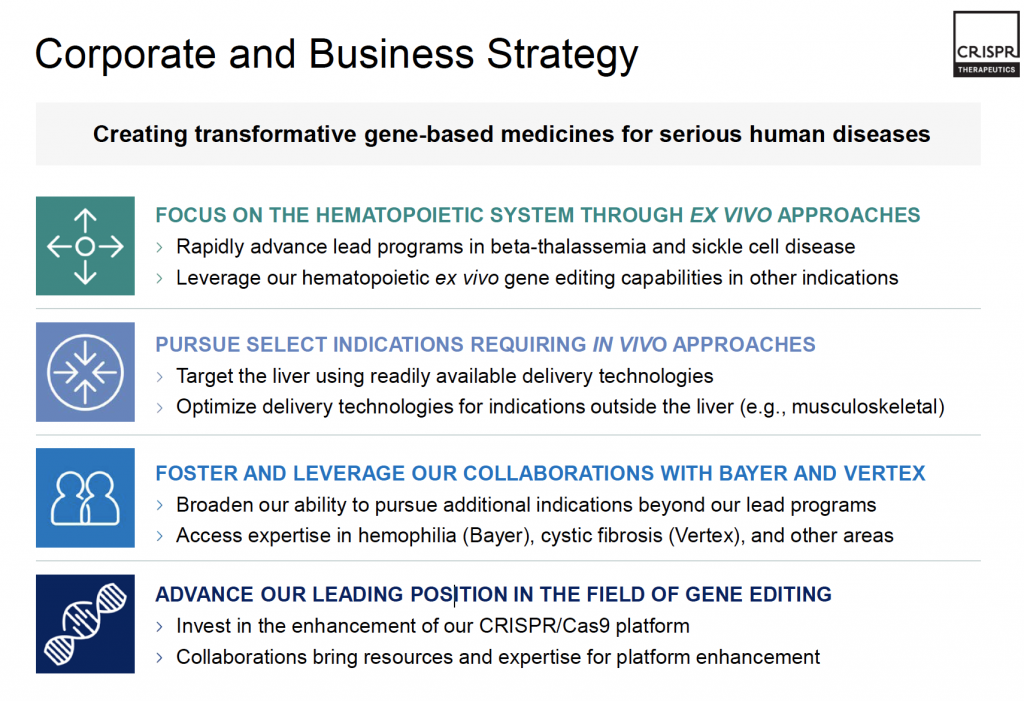
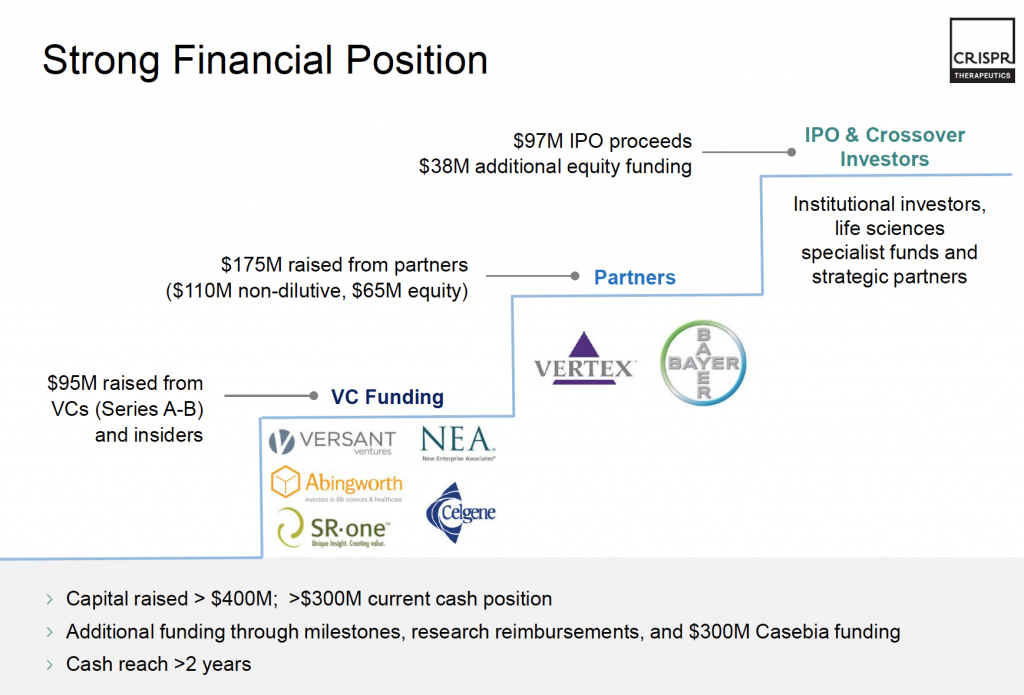
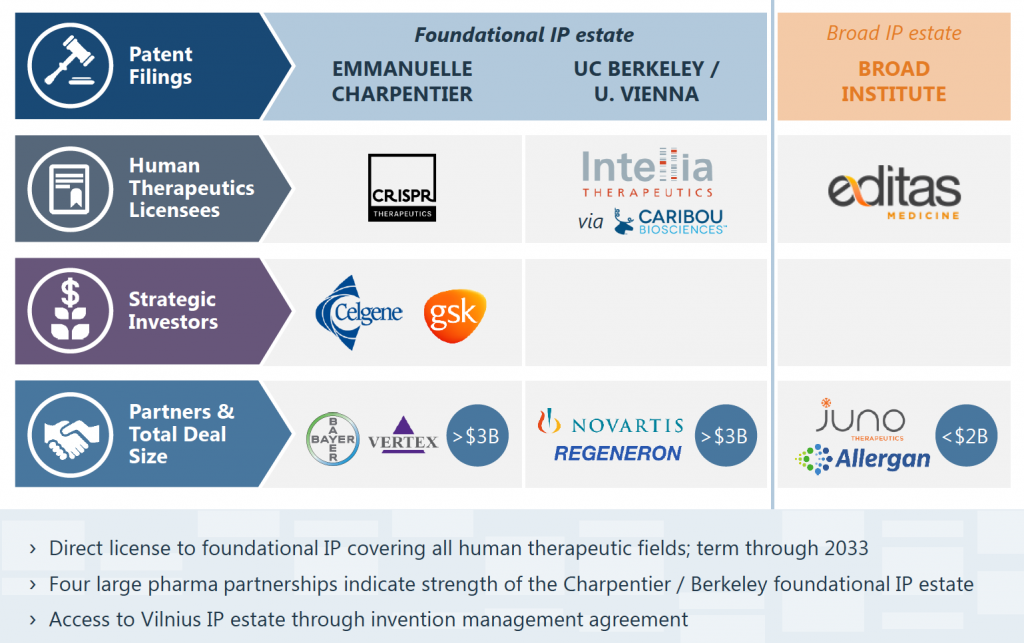
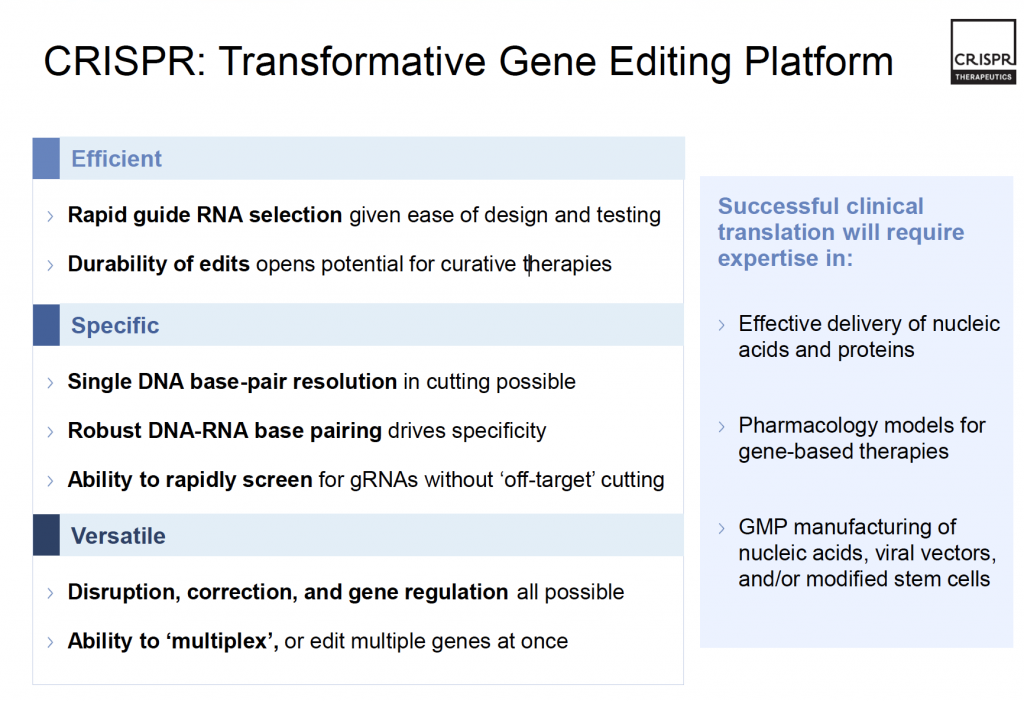
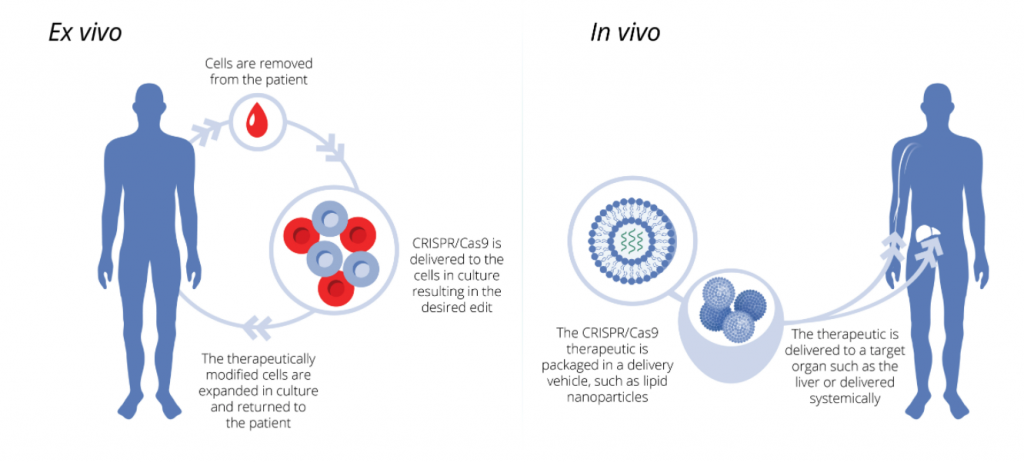
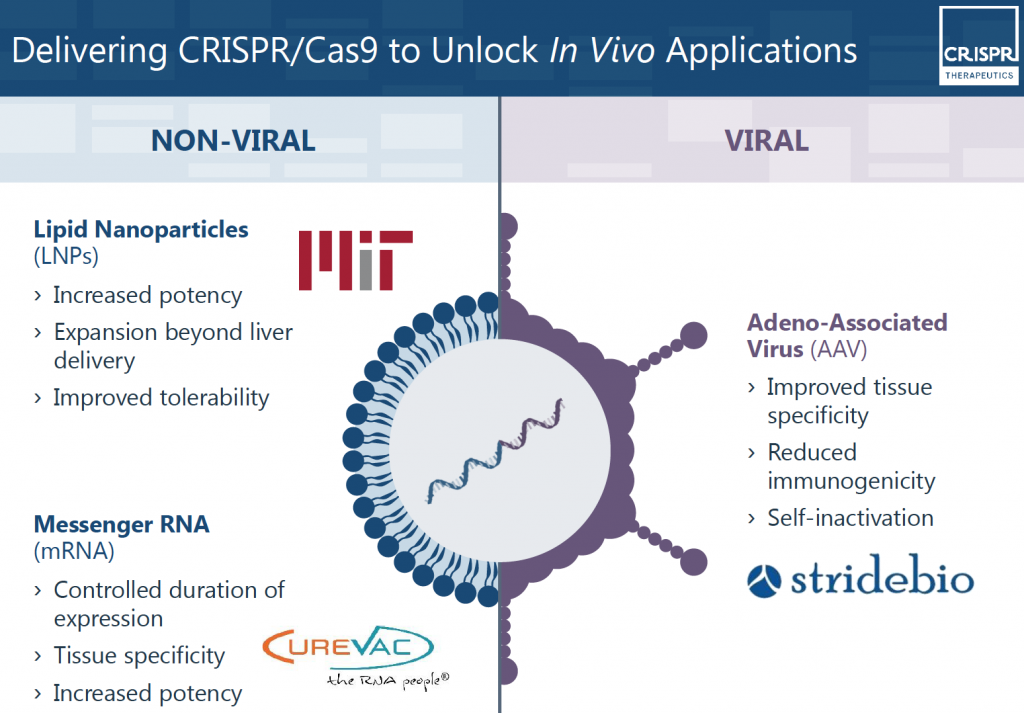
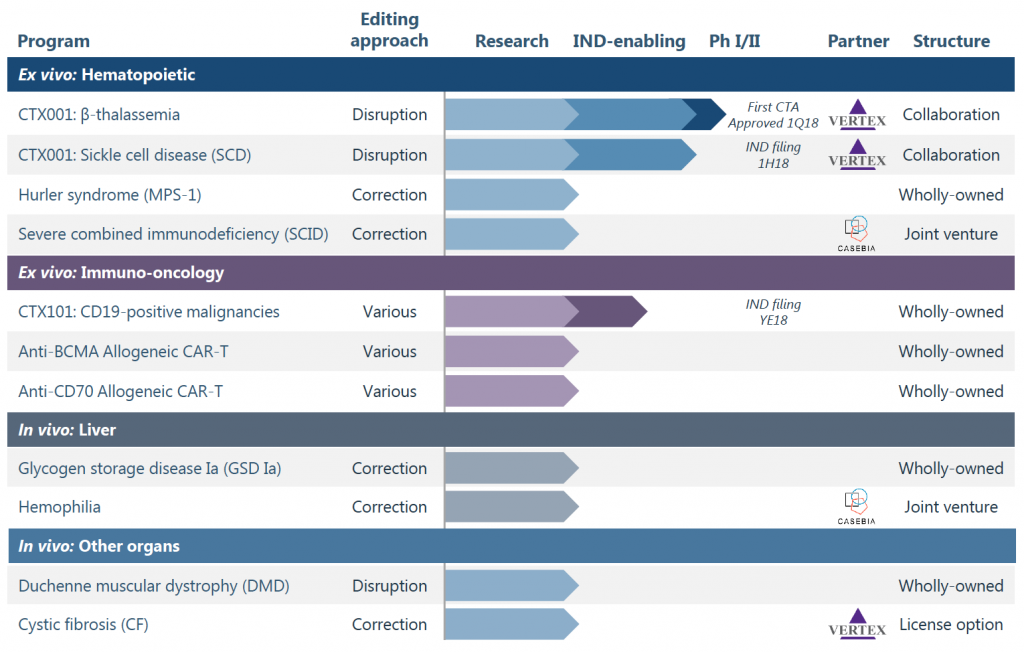