11.9 The Death of a High-Mass Star
If what we have described so far were the whole story of the evolution of stars and elements, we would have a big problem on our hands. We will see in a later chapter that in our best models of the first few minutes of the universe, everything starts with the two simplest elements—hydrogen and helium (plus a tiny bit of lithium). All the predictions of the models imply that no heavier elements were produced at the beginning of the universe. Yet when we look around us on Earth, we see lots of other elements besides hydrogen and helium. These elements must have been made (fused) somewhere in the universe, and the only place hot enough to make them is inside stars. One of the fundamental discoveries of twentieth-century astronomy is that the stars are the source of most of the chemical richness that characterizes our world and our lives.
We have already seen that carbon and some oxygen are manufactured inside the lower-mass stars that become red giants. But where do the heavier elements we know and love (such as the silicon and iron inside Earth, and the gold and silver in our jewelry) come from? The kinds of stars we have been discussing so far never get hot enough at their centers to make these elements. It turns out that such heavier elements can be formed only late in the lives of more massive stars.
Massive stars evolve in much the same way that the Sun does (but always more quickly)—up to the formation of a carbon-oxygen core. One difference is that for stars with more than about twice the mass of the Sun, helium begins fusion more gradually, rather than with a sudden flash. Also, when more massive stars become red giants, they become so bright and large that we call them supergiants. Such stars can expand until their outer regions become as large as the orbit of Jupiter, which is precisely what the Hubble Space Telescope has shown for the star Betelgeuse. They also lose mass very effectively, producing dramatic winds and outbursts as they age. Figure 11.19. shows a wonderful image of the very massive star Eta Carinae, with a great deal of ejected material clearly visible.
Eta Carinae
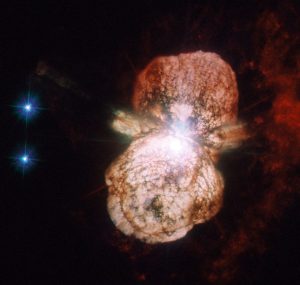
Preview of a forthcoming supernova by ESA/NASA, ESA Standard License.
But the crucial way that massive stars diverge from the story we have outlined is that they can start additional kinds of fusion in their centres and in the shells surrounding their central regions. The outer layers of a star with a mass greater than about 8 solar masses have a weight that is enough to compress the carbon-oxygen core until it becomes hot enough to ignite fusion of carbon nuclei. Carbon can fuse into still more oxygen, and at still higher temperatures, oxygen and then neon, magnesium, and finally silicon can build even heavier elements. Iron is, however, the endpoint of this process. The fusion of iron atoms produces products that are more massive than the nuclei that are being fused and therefore the process requires energy, as opposed to releasing energy, which all fusion reactions up to this point have done. This required energy comes at the expense of the star itself, which is now on the brink of death as shown in Figure 11.20.
Interior Structure of a Massive Star Just before It Exhausts Its Nuclear Fuel
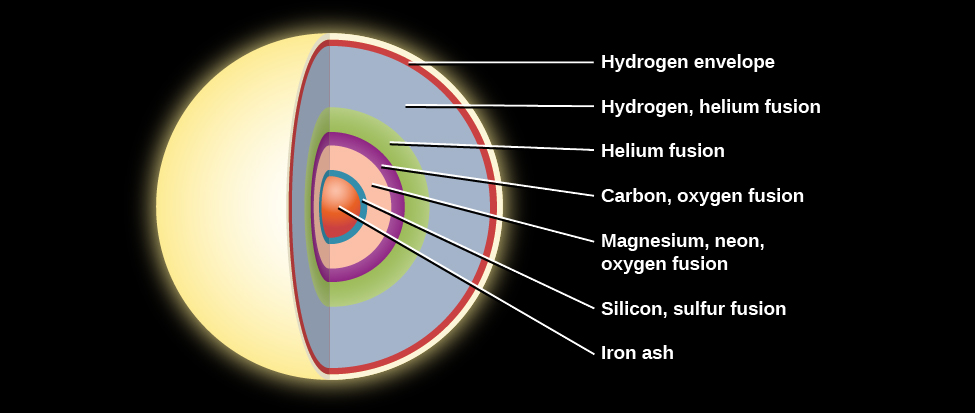
Physicists have now found nuclear pathways whereby virtually all chemical elements of atomic weights up to that of iron can be built up by this nucleosynthesis (the making of new atomic nuclei) in the centres of the more massive red giant stars. This still leaves the question of where elements heavier than iron come from. We will see in the next chapter that when massive stars finally exhaust their nuclear fuel, they most often die in a spectacular explosion—a supernova. Heavier elements can be synthesized in the stunning violence of such explosions.
Not only can we explain in this way where the elements that make up our world and others come from, but our theories of nucleosynthesis inside stars are even able to predict the relative abundances with which the elements occur in nature. The way stars build up elements during various nuclear reactions really can explain why some elements (oxygen, carbon, and iron) are common and others are quite rare (gold, silver, and uranium).
Compared with the main-sequence lifetimes of stars, the events that characterize the last stages of stellar evolution pass very quickly (especially for massive stars). As the star’s luminosity increases, its rate of nuclear fuel consumption goes up rapidly—just at that point in its life when its fuel supply is beginning to run down.
After the prime fuel—hydrogen—is exhausted in a star’s core, we saw that other sources of nuclear energy are available to the star in the fusion of, first, helium, and then of other more complex elements. But the energy yield of these reactions is much less than that of the fusion of hydrogen to helium. And to trigger these reactions, the central temperature must be higher than that required for the fusion of hydrogen to helium, leading to even more rapid consumption of fuel. Clearly this is a losing game, and very quickly the star reaches its end.
Attribution
“22.5 The Evolution of More Massive Stars” from Douglas College Astronomy 1105 by Douglas College Department of Physics and Astronomy, is licensed under a Creative Commons Attribution 4.0 International License, except where otherwise noted. Adapted from Astronomy 2e.