22 The Citric Acid Cycle
“If citrate is added the rate of respiration is often increased… the extra oxygen uptake is by far greater than can be accounted by the complete oxidation of citrate…Since citric acid reacts catalytically in the tissue, it is probably removed by a primary reaction and then regenerated by a subsequent reaction.” -H. A. Krebs and W. A. Johnson, article in Enzymologia, 1937
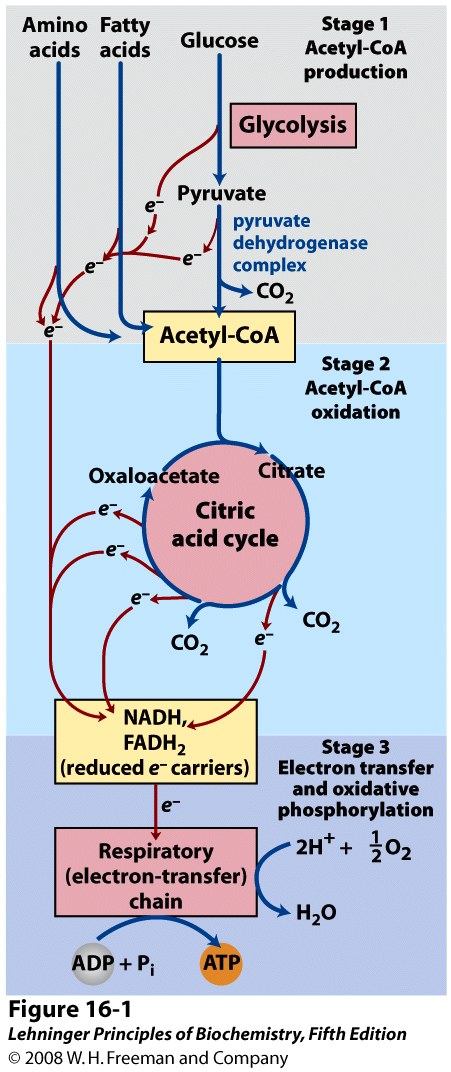
We have discussed beta oxidation and glycolysis. These are the first stages of fatty acid and glucose catabolism, respectively. Some organisms and certain cells obtain energy exclusively through the anaerobic breakdown of glucose to lactate or ethanol. However, most eukaryotic cells obtain their energy through the complete oxidation of organic fuels into CO2 and H2O through aerobic metabolism. This aerobic phase of catabolism is known as respiration. Although we usually identify respiration as the uptake of O2 and release of CO2 by the respiratory system during breathing, biochemists use the term to refer to the molecular processes through which cells consume O2 and produce CO2: processes more specifically referred to as cellular respiration.
The Citric Acid cycle constitutes the second stage of cellular respiration (Figure 22.1). It takes the acetate (two-carbon) moieties derived from the catabolism of fats, carbohydrates and certain amino acids (in the form of acetyl-CoA) and oxidizes them completely to carbon dioxide; the energy released through these oxidations is conserved in the form of reduced cofactors, NADH and FADH2. The reduced cofactors in turn are oxidized by the mitochondrial electron transport chain with molecular O2 acting as the ultimate oxidant (discussed in Chapter 23); the released energy is captured to form ATP through the process known as oxidative phosphorylation (Stage 3 of catabolism). The citric acid cycle also supplies or consumes many important intermediates derived from/converted into amino acids, nucleotides and other key materials.
Discovery of the TCA cycle
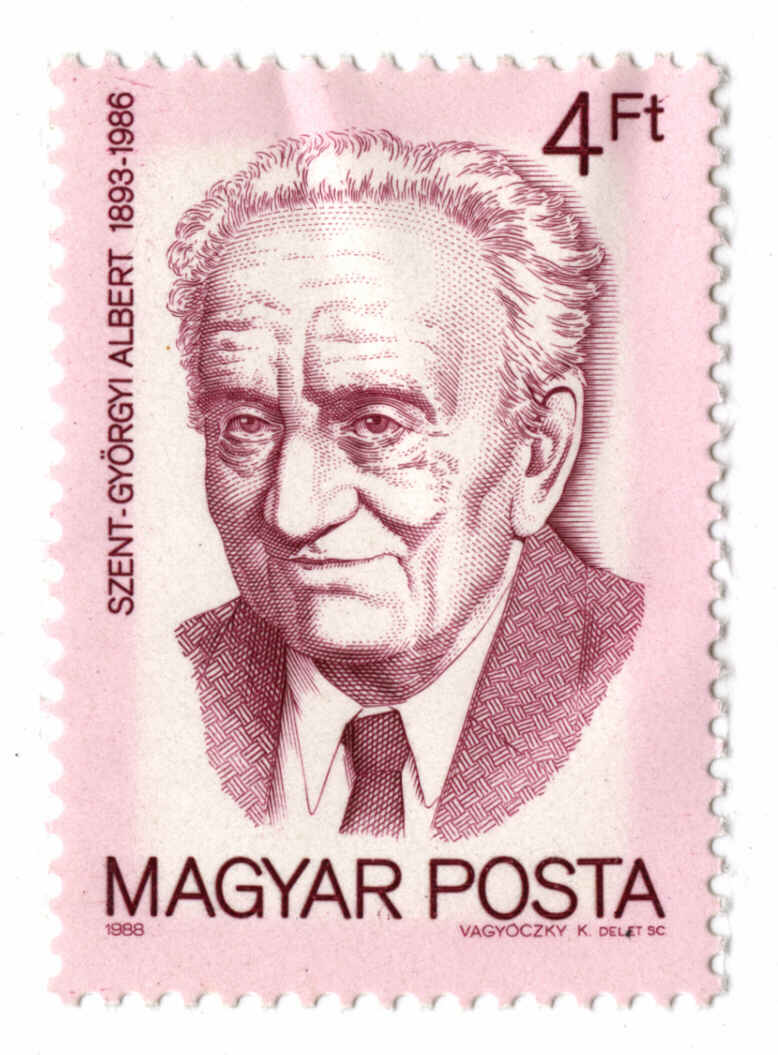
The great Hungarian biochemist Albert Szent-Györgyi (who won the Nobel Prize in 1937 for the discovery of vitamin C, Figure 22.2) was one of the pioneers whose studies led to the concept of the citric acid cycle. This was back in the 1930s. Szent-Györgyi made preparations from an actively-respiring tissue (pigeon flight muscle) and measured their respiration rate, by using an old-fashioned manometer gauge to measure their consumption of oxygen. He found that if he added a little bit of certain organic acids such as succinic acid to the tissue preparation it would stimulate a burst of respiration, larger than could be explained by the oxidation of the added succinic acid. The acids acted catalytically, not stoichiometrically. This was an important clue!
Another important clue came from studies of the metabolism of citric acid. Citric acid is the acid abundant in citrus fruits like lemons and oranges. Citric acid was found to be oxidized to a compound called alpha-ketoglutarate. This was discovered by Knoop – the same scientist who discovered beta-oxidation, as we have noted earlier.
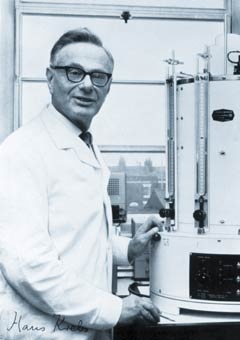
But the person who really deserves the credit for figuring out the citric acid cycle is Hans Krebs (Figure 22.3); he won the Nobel Prize in 1953 (sharing it with Fritz Lipmann, whom we have mentioned earlier). Krebs was another scientist who fled Germany after Hitler seized power; his work on the citric acid cycle was done at the University of Sheffield in England. One of the discoveries that helped to unravel the citric acid cycle was the finding that malonate blocked respiration leading to the accumulation of succinate. Krebs realized that malonate was acting as a competitive inhibitor of an enzyme (now known as succinate dehydrogenase) that catalyzes succinate oxidation. Citrate was also found to be a product of oxaloacetate. Krebs had the breakthrough idea that the metabolism of all these organic acids was linked together in a cyclic, rather than linear, pathway. By 1937, Krebs had put the puzzle together.
By about 1940, most of the intermediates of the citric acid cycle were understood and it could be written down in almost the same way that you see it in the textbooks today. However, the involvement of acetyl-CoA was not appreciated until after 1945, when Lipmann discovered this co-factor (Krebs had assumed that pyruvate was the feedstock for the cycle). The Krebs cycle was one of the last major developments in biochemistry made before the use of radioisotopes.
As stated earlier, the citric acid cycle effects the complete oxidation of acetate (CH3-COO–) to CO2. It is a complicated sequence of transformations, beginning with the incorporation of acetate into a six-carbon compound, citric acid. Why not just oxidize acetate directly to CO2? There are probably several reasons. Firstly, the alpha carbon (methyl group) of acetate is not very “activated”; it would be chemically difficult to oxidize it directly. In the citric acid cycle, this carbon becomes linked to a beta carbon atom, allowing it to be oxidized by the same mechanism that we saw operating in fatty acid beta oxidation. Secondly, the citric acid cycle was undoubtedly derived from more ancient, non-oxidative metabolic processes of anaerobic cells; the aerobic citric acid cycle was “cobbled together” after the evolution of the “aerobic lifestyle”. So it does not necessarily follow the simplest possible route to CO2. Thirdly, although the intermediacy of several metabolites (citrate, succinate, oxaloacetate, etc.) between acetate and carbon dioxide makes the metabolic process more complicated, it also provides multiple opportunities for interfacing the citric acid cycle with other pathways of catabolism and anabolism.
The TCA cycle consists of 8 steps.
Step 1: Condensation of oxaloacetate with acetyl-CoA
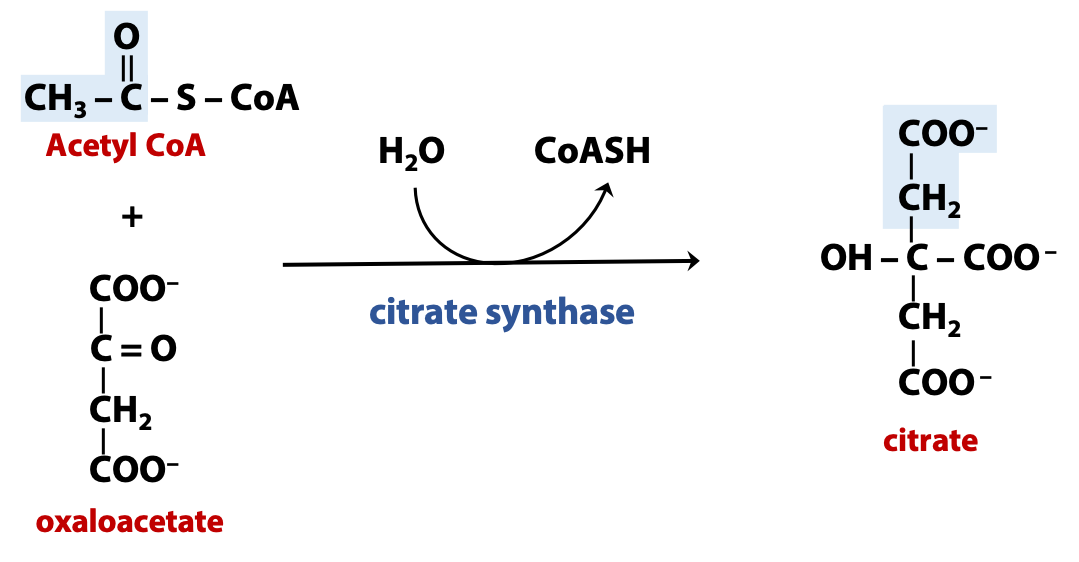
The citric acid cycle starts with the condensation of acetate with oxaloacetate to form the tricarboxylic acid, citric acid (Figure 22.4). Acetate enters the cycle as acetyl-CoA and free coenzyme A is liberated in the reaction. The enzyme catalyzing the reaction is citrate synthase.
The methyl carbon of acetyl-CoA, once deprotonated by the citrate synthase enzyme, acts as the nucleophile in this reaction. It attacks the electrophilic carbonyl carbon of oxaloacetate. Citrate synthase is the only step of the TCA cycle which a C-C bond is formed.
Step 2: Formation of isocitrate through cis-aconitate
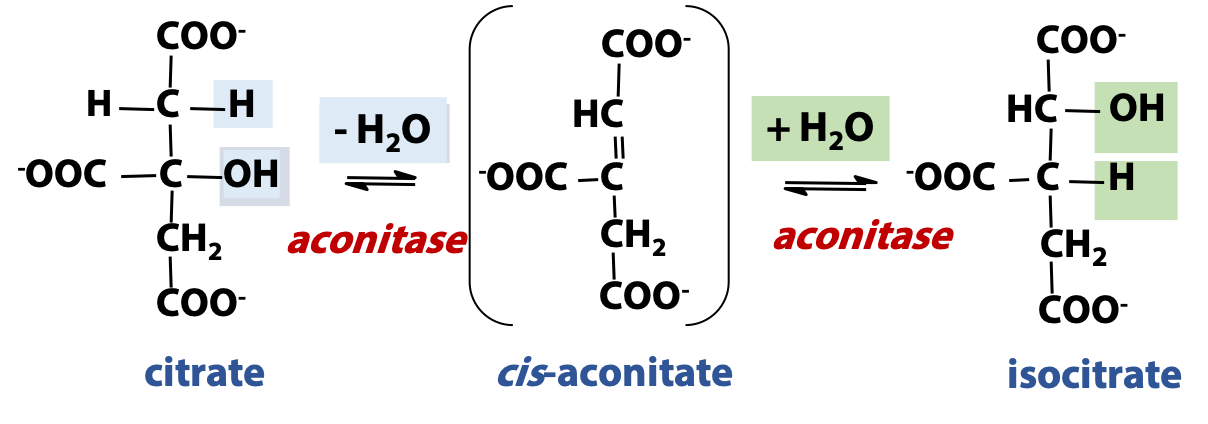
Citrate is a tertiary alcohol, and these are not readily oxidized. An isomerization converts citrate to an isomer, isocitrate (Figure 22.5). This is a secondary alcohol and is more easily oxidized. The isomerization proceeds by the reversible hydration of an alkene, cis-aconitic acid (cis-aconitate). Hydration in one orientation gives citrate; hydration in the opposite orientation gives isocitrate. This reaction is close to equilibrium, in terms of free energy change, but the consumption of isocitrate in the next step of the cycle drives the isomerization step forward. The enzyme catalyzing the isomerization is called “aconitase”.
Step 3: Oxidation of isocitrate to alpha-ketoglutarate and CO2
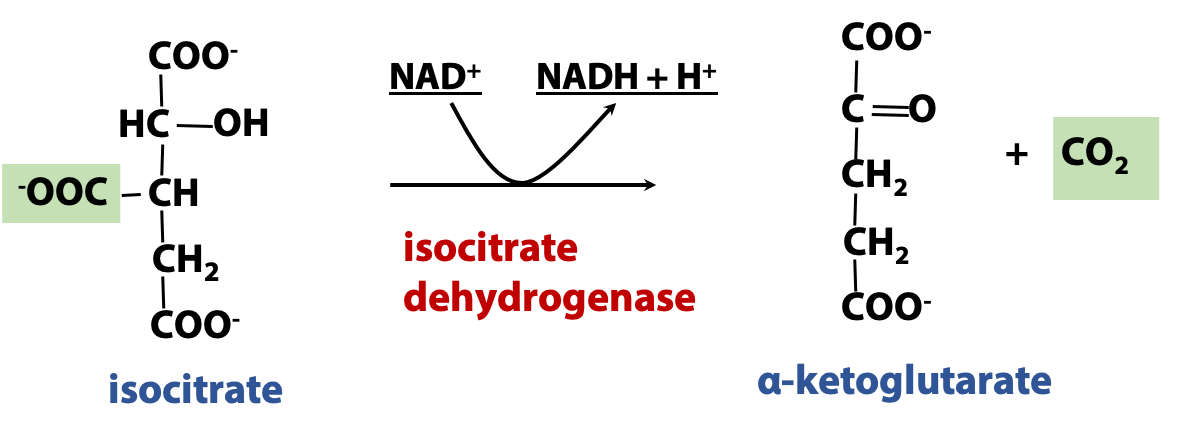
The next step of the citric acid cycle is an oxidative decarboxylation catalyzed by isocitrate dehydrogenase which converts isocitrate to alpha ketoglutarate (Figure 22.6). (If you replace the keto group of -Ketoglutarate by an amino group, you have the amino acid glutamate. Indeed, this interconversion occurs easily in the cell; it is catalyzed by enzymes called “transaminases”.)
This reaction involves the oxidation of the alcohol group of isocitrate to a ketone through the transfer of a hydride from the C-H of the alcohol to NAD+ followed by the loss of the –COO group as CO2 (oxidative decarboxylation).
Step 4: Oxidation of -Ketoglutarate to succinyl CoA and CO2
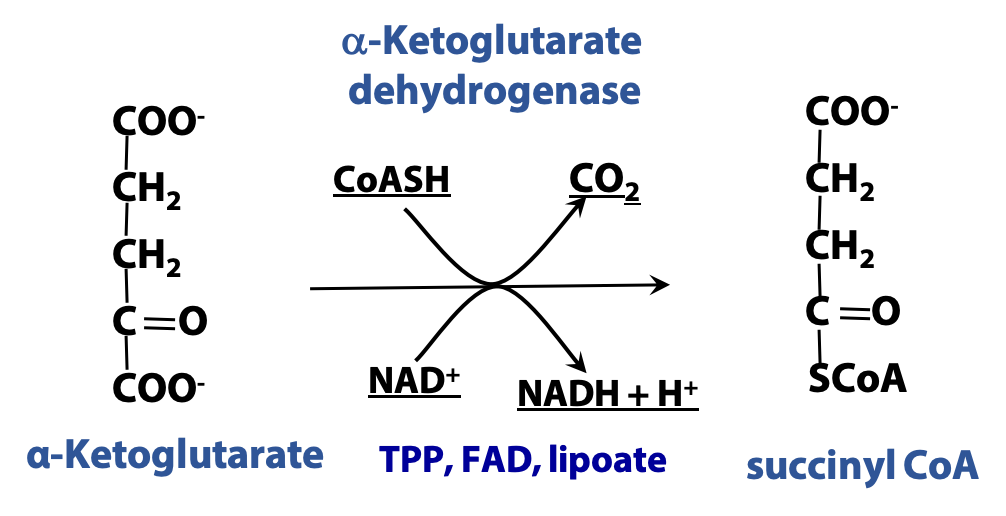
α-ketoglutarate dehydrogenase, catalyzes another oxidative decarboxylation, but the mechanism is different from that of isocitrate dehydrogenase. (In fact, this reaction is very similar to the pyruvate dehydrogenase-catalyzed oxidation of pyruvate to acetate and CO2. This reaction connects glycolysis to the citric acid cycle. Pyruvate dehydrogenase and α-ketoglutarate dehydrogenase are different, but closely related enzymes). This reaction converts α-ketoglutarate to the high-energy thioester succinyl-CoA (Figure 22.7).
Step 5: Conversion of succinyl CoA to succinate
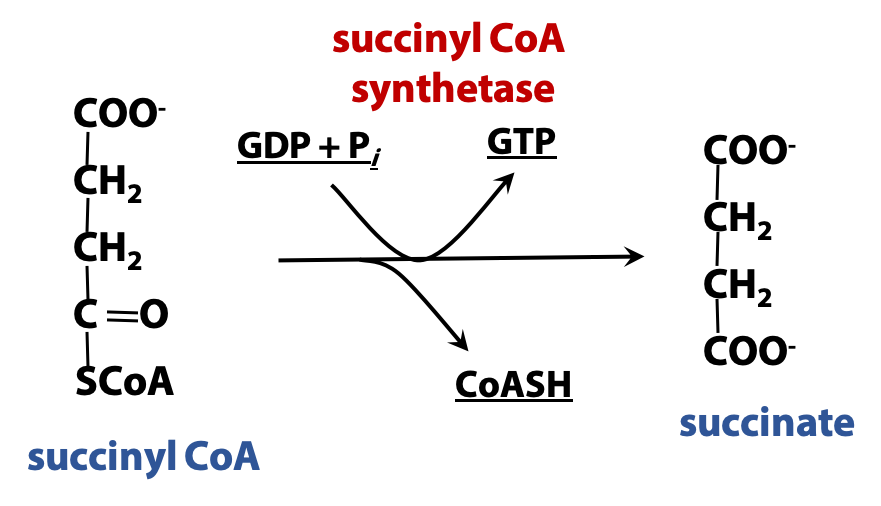
The high-energy thioester bond of succinyl CoA is hydrolyzed in a reaction coupled to ATP synthesis, catalyzed by succinyl CoA synthetase (Figure 22.8). Synthetases are ATP-dependent synthases (enzymes that join two molecules into one). This enzyme (like many others) is named for a reaction that is the reverse of the usual physiological process; usually, acting to form succinate from succinyl CoA. So, in this step, energy is conserved as ATP. (There are two forms of this enzyme; one generates ATP and the other generates GTP; GTP is a high-energy compound, just like ATP and GTP energy can be exchanged “one-for-one” with ATP energy.)
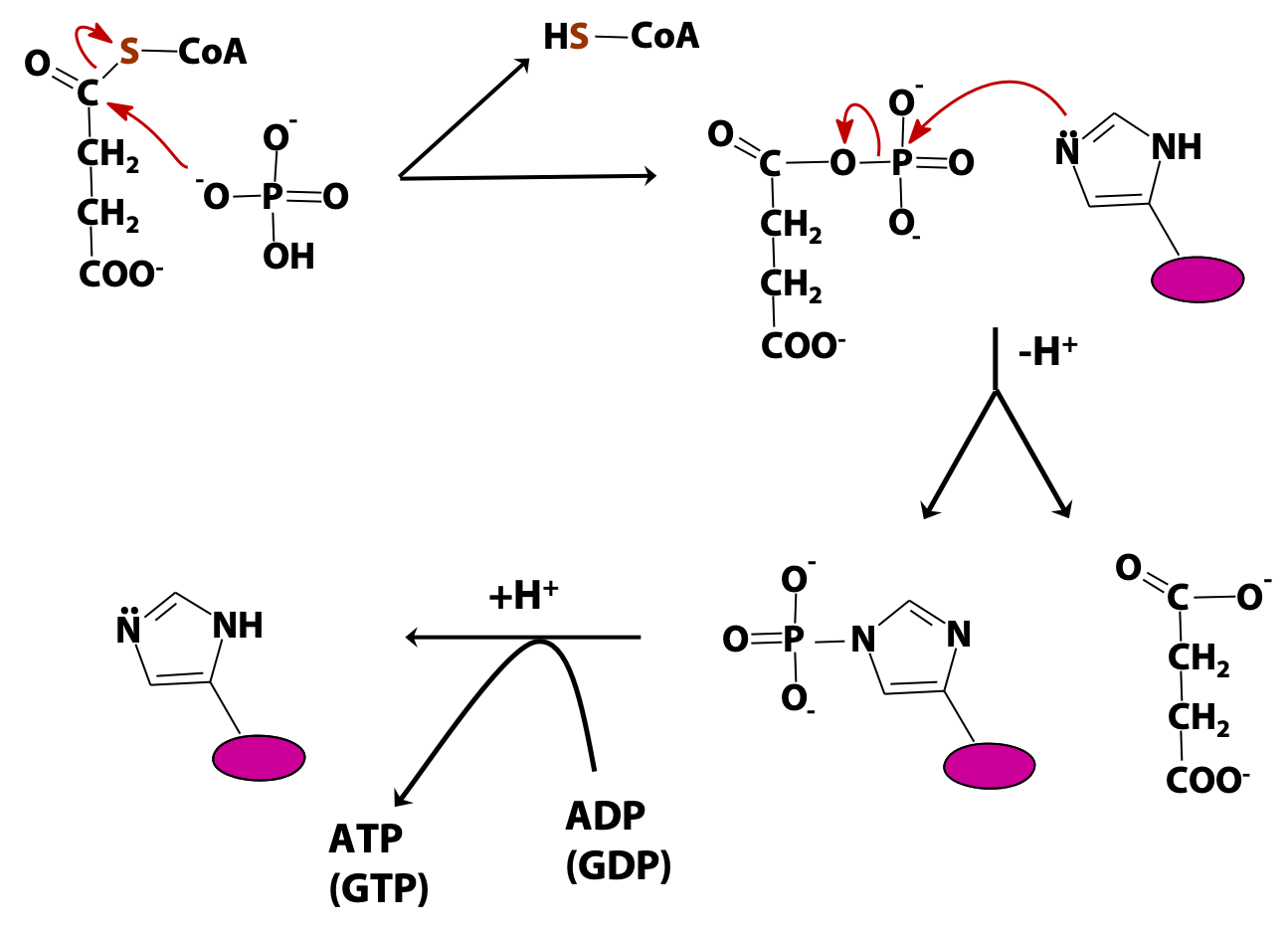
Succinyl CoA synthetase generates ATP (or GTP). This is an example of “substrate-level phosphorylation” (i.e., the direct formation of a high-energy phosphate from an energy-rich metabolite, as opposed to oxidative phosphorylation, which we will study soon). The mechanism is shown in Figure 22.9 . In the first step, inorganic phosphate displaces coenzyme A from succinyl CoA, forming a high-energy acyl phosphate, succinyl phosphate. Next, a reactive histidine residue at the active site of the enzyme accepts the phosphate group, forming a relatively long-lived phosphoenzyme intermediate and releasing succinate. Finally, the phosphate is transferred from the enzyme to the ADP (or GDP), releasing the free enzyme.
Step 6: Oxidation of succinate to fumarate
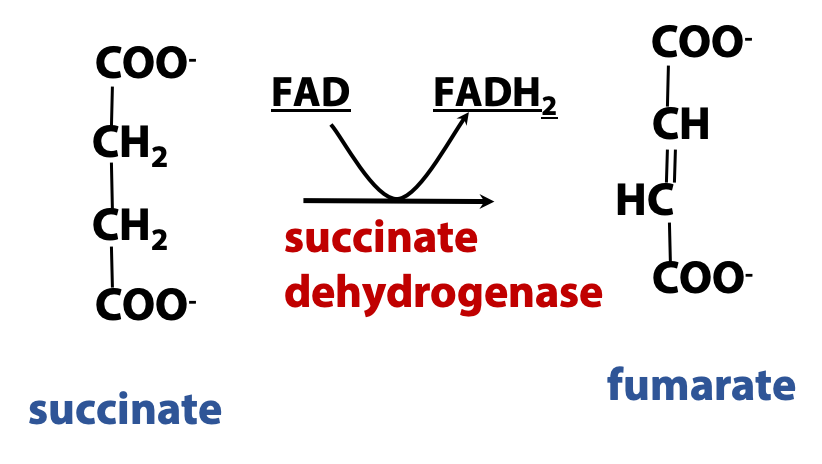
Succinate is a four-carbon compound, just like oxaloacetate, except that it has a methylene group, while oxaloacetate has a ketone. Therefore, in order to complete the cycle, we have to oxidize succinate back to oxaloacetate, by oxidizing the … yes, that’s right, the beta carbon (Figure 22.10)! (Well, it’s beta with respect to one of the two carboxylic acid groups in succinate, anyway; succinate is symmetrical). If you think that this is starting to sound like beta oxidation, you’re right: it’s completely analogous. The enzymes are different, but the mechanisms are the same. So you will see that even though we have barely begun the study of metabolism, we are already starting to observe common themes among apparently unrelated processes. Succinate dehydrogenase uses FAD to oxidize succinate to the alkene fumarate. This reaction is analogous to the acyl-CoA dehydrogenase reaction of beta oxidation seen in Chapter 20.
Step 7: Hydration of fumarate to malate
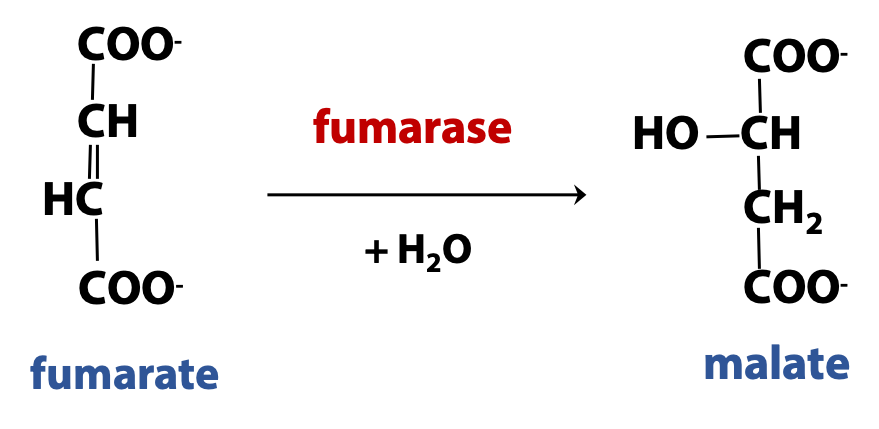
The alkene, fumarate, is hydrated to the alcohol, malate (malic acid) (Figure 22.11). This reaction is analogous to the enoyl-CoA hydratase reaction of beta oxidation.
Step 8: Oxidation of malate to oxaloacetate
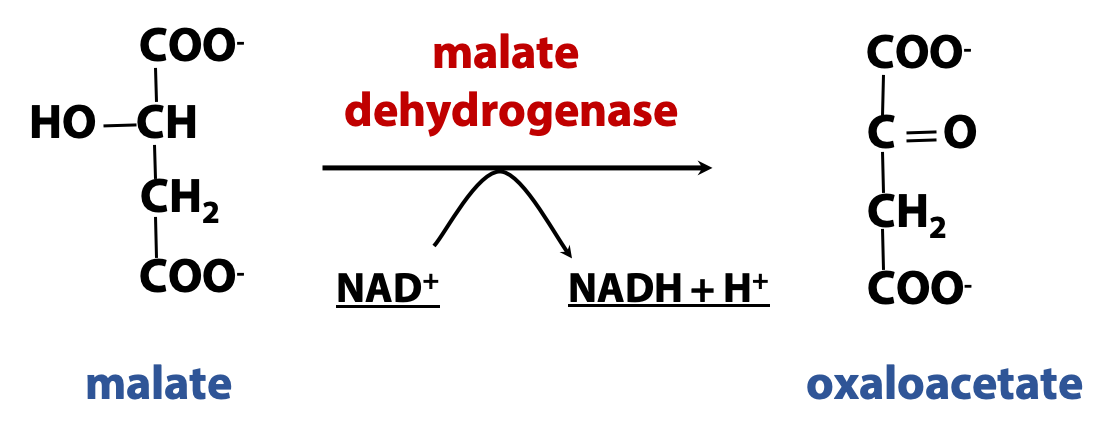
In the final step of the cycle, malate is oxidized to oxaloacetate by NAD+ (Figure 22.12). Now we are back at the beginning of the citric acid cycle. This step is analogous to the hydroxyacyl-CoA dehydrogenase reaction of beta oxidation.
Note that oxaloacetate is the four-carbon alpha-keto acid corresponding to the four-carbon alpha-amino acid, aspartate. This relationship is the same as the relationship between α-ketoglutarate and glutamate. So you can guess that the catabolism of aspartate and glutamate links to the citric acid cycle.
The overall stoichiometry of the citric acid cycle per turn:
The citric acid cycle cannot serve as a net source of (or a sink for) its intermediates (citrate, succinate, etc.); each of these intermediates is formed and then consumed with each turn of the cycle. In terms of effect, they act more like catalysts than substrates, as Szent-Györgyi appreciated from the outset. Each round of the citric acid cycle converts the equivalent of one acetyl-CoA into two molecules of carbon dioxide, liberating one CoASH. One ATP (or GTP) is formed at the succinyl CoA synthetase step. The major products of the citric acid cycle, in terms of bioenergetics, are reduced cofactors, 3 NADH and 1 FADH2 per turn. As we will see next, the reoxidation of these reduced cofactors generates a very large amount of ATP.
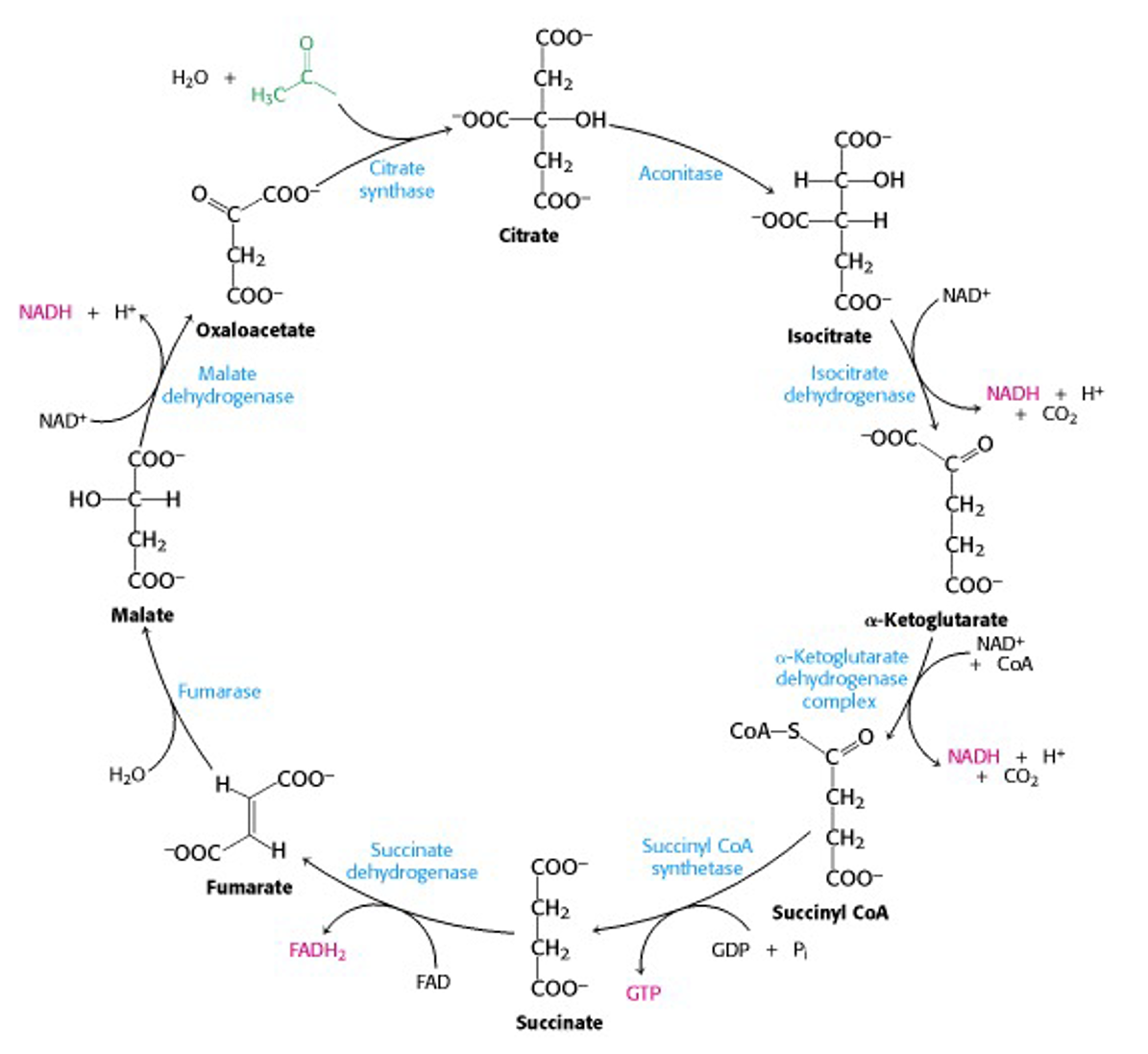
So, here’s the Krebs cycle in full (Figure 22.13). The input acetyl-CoA is in green while the output reduced units of NADH and FADH2 are in pink. Note where CO2 is released.