23 Oxidative Phosphorylation: The Electron Transport Chain
Oxidative phosphorylation constitutes the final and 3rd stage of oxidation of carbohydrates, lipids and amino acids. During this process, energy of oxidation is used to synthesise ATP. It occurs in the mitochondria and begins with the oxidation of reduced cofactors, NADH and FADH2 by the electron transport chain.
Reduced cofactors such as NADH and FADH2 are good reducing agents. Oxygen is a very good oxidizing agent – one of the strongest known to chemistry. So the oxidation of any kind of reduced carbon compound is highly favourable thermodynamically. Now we see why oxygen presented such a threat – and such an opportunity – to life on this planet, when it first began to accumulate in the atmosphere, billions of years ago, thanks to photosynthesis. Oxygen not only provided a vast new energy source, but it also presented the danger of uncontrolled oxidation processes (not only fires, but also chemical chain reactions like lipid peroxidation, which is what happens when you leave butter outside the refrigerator for too long in the summer and it gets rancid).
Oxidation of reduced cofactors: energetics
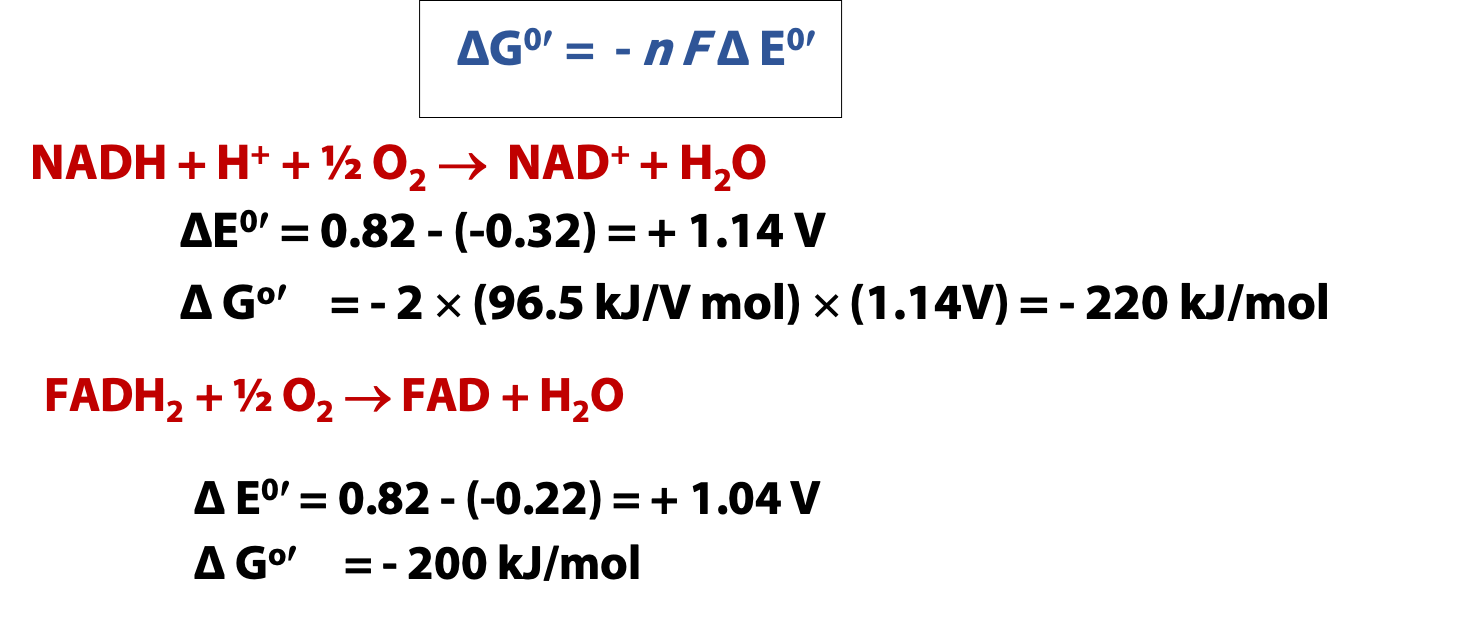
The calculations in Figure 23.1 show you how much energy is released by the reoxidation of NADH and FADH2. Since oxygen is such a good oxidizing agent, both reactions have very large negative Gibbs free energy changes. NADH is a little stronger, as a reducing agent, so its free energy change is a bit larger. But both numbers are much larger than any covalent bond strength. So, the reoxidation of one mole of reduced cofactor liberates enough energy to make several moles of ATP. IF the energy of the redox reaction can be harnessed to ATP production!
However, so much energy is released by the reaction between the reduced cofactors and oxygen that the direct reaction, even if it were catalyzed, would be energetically wasteful: no covalent bond could contain more than a fraction of the energy released. So, instead, nature uses electron transport chains. The reducing equivalents** from the reduced cofactors are passed to oxygen indirectly. So, instead of releasing all the available energy at once, the reoxidation of NADH and FADH2 by oxygen is broken up into several distinct processes with smaller free energy changes. This is just like lowering ourselves gently from floor to floor (in an elevator) when moving from the fifth floor of a building to the first; for, although jumping out the fifth floor window is an effective way to get to the ground, it is dangerous and the process releases so much energy.
**Three types of electron transfers occur in the electron transport chain;
- Direct transfer as in the reduction of Fe3+ to Fe2+
- Transfer of a hydrogen atom (H+ + e–)
- Transfer of a hydride ion (:H–) bearing two electrons
We use the term reducing equivalent to signify electrons transferred in all these types of transfers.
In an electron transport chain, we pass reducing equivalents from molecule to molecule, always moving in the direction of higher reduction potential, until we get to oxygen, the “terminal electron acceptor” (that is, the oxidant with the most positive reduction potential). To accomplish this, we need to have a special set of electron carriers, molecules with appropriate reduction potentials, intermediate between NADH and oxygen.
There are many different electron transport chains in biology. For example, E. coli has a quite different chain from the eukaryotic mitochondrion (the chain we are going to look at in the most detail). But the general principles are always the same.
Mitochondrial Electron transport Chain
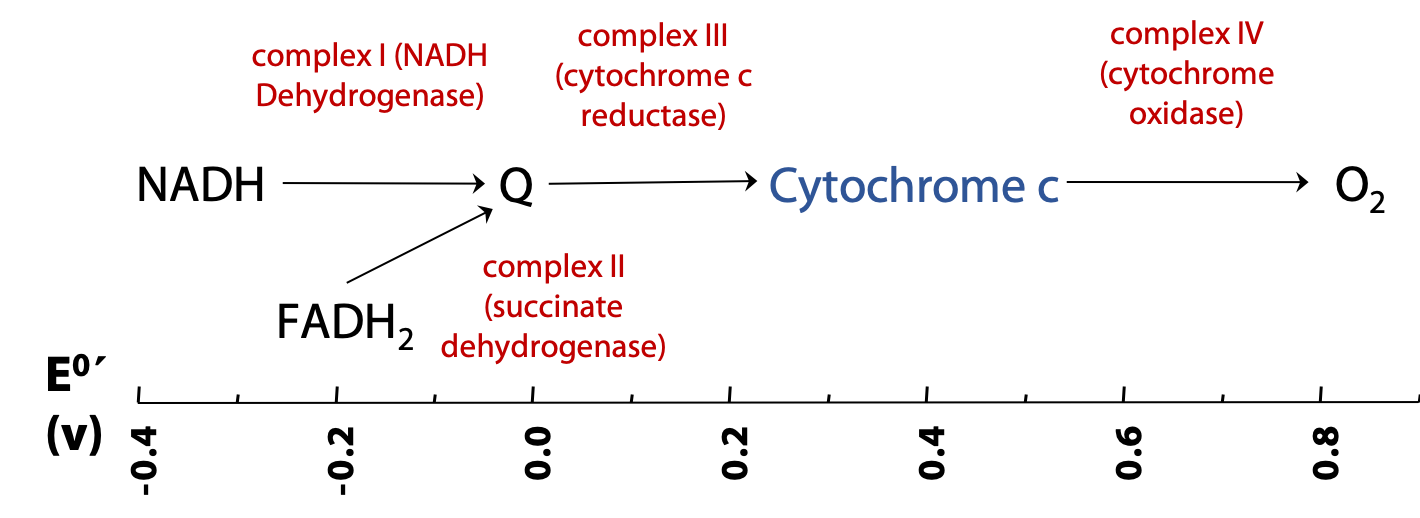
Figure 23.2 shows the overall plan of mitochondrial electron transport. This is a highly simplified plan; in reality, each of the complexes noted here is a very elaborate system of proteins and associated redox-active species, such as cofactors and metal ions. Reducing equivalents from NADH are passed to a called coenzyme Q; and then to the small heme-protein cytochrome c; and finally to oxygen. Each of these transfers is catalyzed by a “complex”. A complex is a big enzyme: an assembly of proteins and other molecules.
FADH2 is oxidized along a convergent pathway, but its reducing equivalents enter the chain a little lower down, since it is a weaker reducing agent than NADH. Complex II is really just succinate dehydrogenase of the citric acid cycle, which we have already seen. Complex II then passes its reducing equivalents to coenzyme Q.
The electron carriers of the Electron Transport chain: Coenzyme Q (ubiquinone)
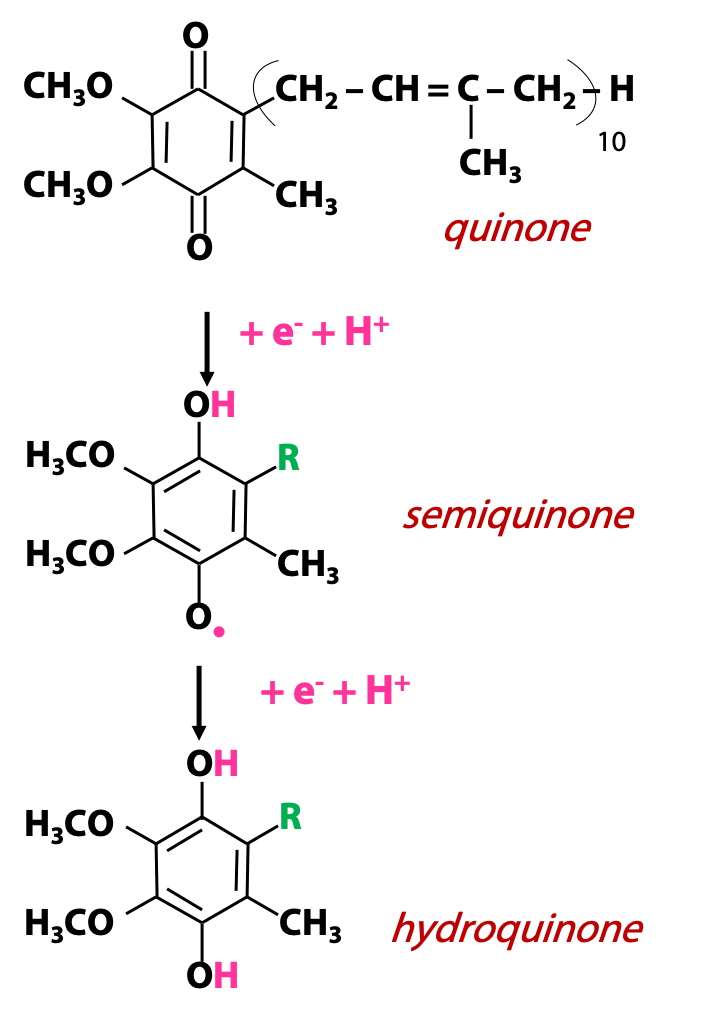
The Q in coenzyme Q stands for quinone. A quinone is an organic functional group with two ketone oxygens in a conjugated six-membered ring. Quinones are oxidizing agents and they become reduced to hydroquinones, as shown. This is a two-electron process accompanied by two protons, and it goes via distinct one-electron steps, so there is a free radical intermediate called a “semiquinone”(·QH). The fully reduced hydroquinone form of coenzyme Q is called ubiquinol (QH2) (Figure 23.3). Coenzyme Q bears a long hydrophobic “tail” (built from “isoprene” units) as one of the substituents on the ring, and this tail keeps the molecule associated with the membrane. It can freely move in the membrane carrying electrons from one ETC complex to another.
Cytochrome C
Cytochromes are a large class of heme proteins participating in redox reactions. Their name is Greek for “coloured stuff in the cell”; they were first identified and classified based on their colours (optical absorption spectra). The respiratory electron transport chain involves several cytochromes, notably the small protein cytochrome c. In cytochrome c, the heme ring is covalently linked to the polypeptide via cysteine residues (Figure 23.4), but not all hemeproteins have covalently-linked heme; in some cases, such as hemoglobin, the heme is bound non-covalently. (See Stryer Fig. 18.16)
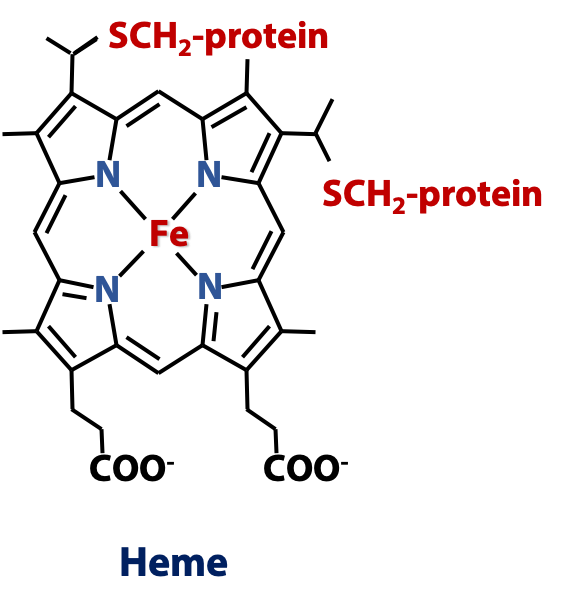
Heme is a prosthetic group (non-peptide component) of many proteins – hemeproteins, and it is a particular example of a larger class of molecules called porphyrins, all of which have similar ring structures. The heme ring joins four pyrrole rings (five-membered rings with one N atom) together into a tetrapyrrole ring, which forms a large conjugated aromatic system. The four N atoms are positioned to form a pocket that can bind a metal ion. In heme, that metal is iron. Heme proteins perform a multitude of biological roles, but they always involve transition metal chemistry – ligands binding to the iron atom or redox processes.
Cytochrome c is a soluble protein in the mitochondrial intermembrane space and shuttles electrons from complex III of the ETC to complex IV. The iron atom of heme acts as the redox active component and carries one electron at a time.
Cytochrome C (Fe3+) + e → Cytochrome C (Fe2+)
The complexes are enzymes that catalyze individual electron-transfer steps in the chain. We can refer to the complexes by their enzyme activities (e.g., NADH:ubiquinone oxidoreductase), but mostly we just refer to them by number: I, II, III, and IV. The complexes are integral membrane proteins; they are buried deeply in the inner mitochondrial membrane. If we gently disrupt the membrane, we can solubilize and purify the individual complexes and measure their catalytic (electron-transfer) activities in the test tube.
Complex I (NADH dehydrogenase):
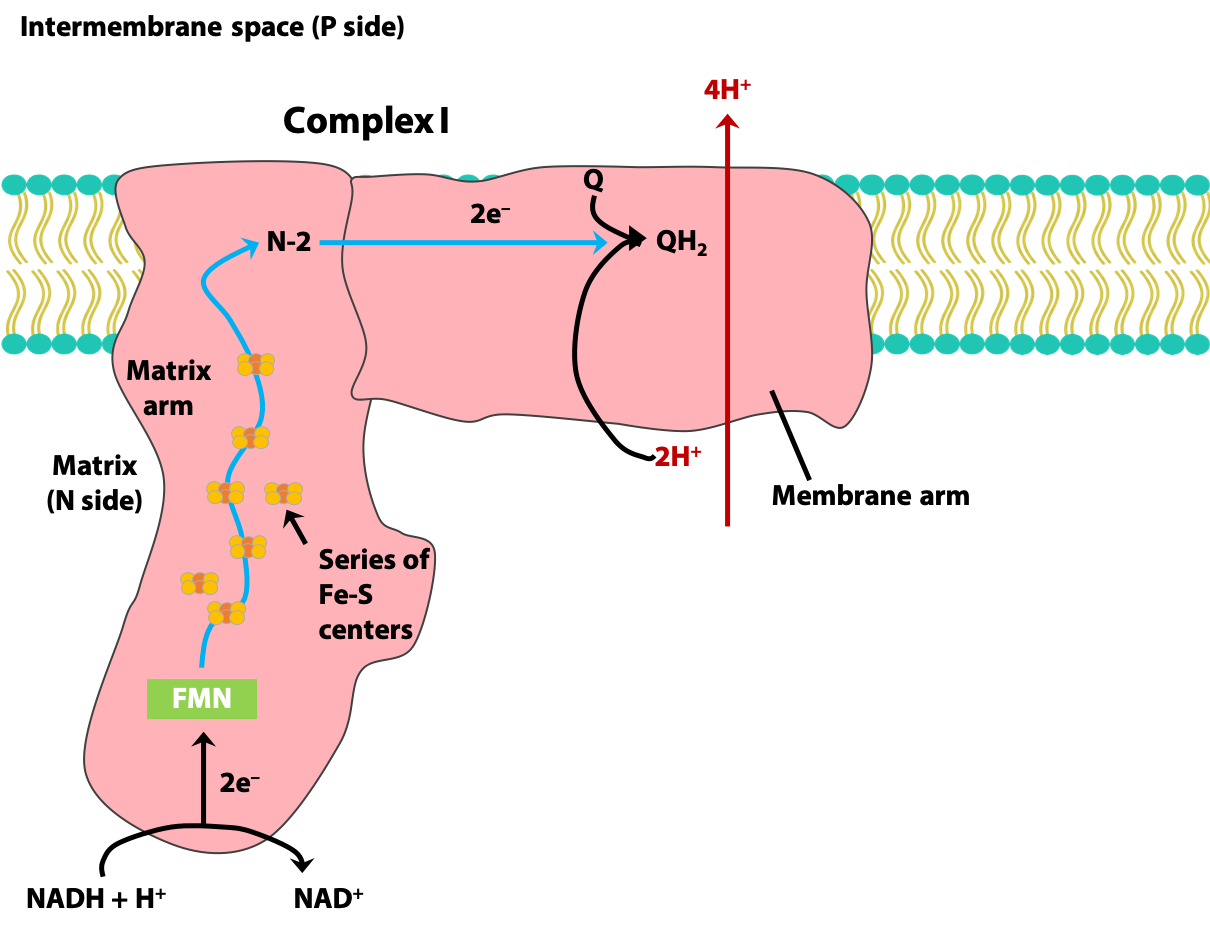
Complex I catalyzes two processes which are obligately coupled to each other (Figure 23.5).
1. Transfer of a hydride ion from NADH and a H+ from the matrix to coenzyme Q (Exergonic)
NADH + H+ + Q → NAD+ + QH2
2. Transfer of four H+ from the matrix to the intermembrane space (Endergonic)
NADH + 5H+N + Q → NAD+ + QH2 + 4HP
(N: matrix side of the inner mitochondrial membrane;
P: the intermembrane space side of the inner mitochondrial membrane)
Complex II (Succinate dehydrogenase):
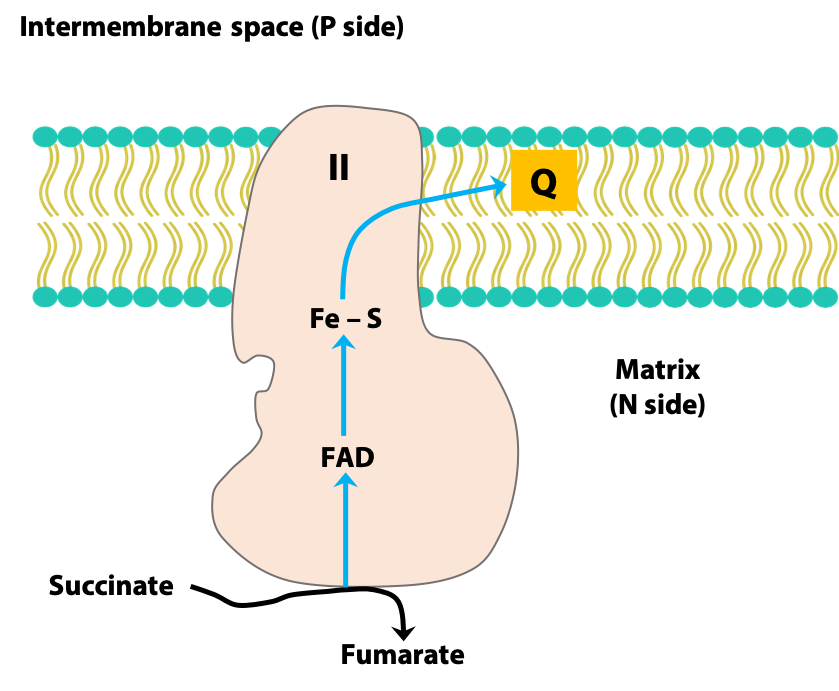
Complex II is the TCA cycle enzyme succinate dehydrogenase. It transfers electrons from FADH2 generated by this reaction to coenzyme Q (Figure 23.6). It does NOT pump protons from the matrix to the intermembrane space.
Complex III (Cytochrome c reductase):
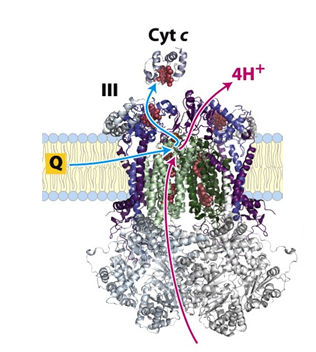
Complex II transfers electrons from QH2 to cytochrome c with the simultaneous transfer of four protons from the matrix to the intermembrane space.
Since each cytochrome c can carry only one electron, oxidation of one mole of QH2 requires two moles of oxidized cytochrome c.
The redox reaction:
QH2 + 2 Cyt C (oxidized) → Q + 2 Cyt C (reduced) + 2 H+
The net equation for Complex III:
QH2 + 2 Cyt C (oxidized) + 2 H+N → Q + 2 Cyt C (reduced) + 4 H+P
Complex IV (Cytochrome oxidase):
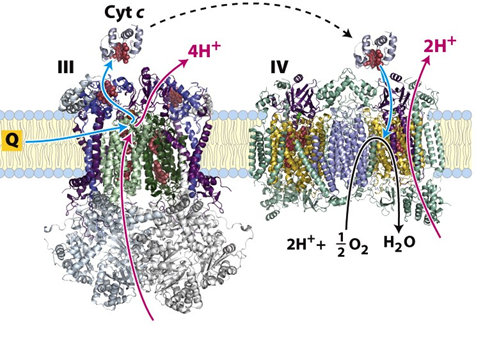
Catalyzes the transfer of electrons from reduced Cyt c to molecular O2, the final electron acceptor. The energy released by the transfer of each 2 electrons is used to pump 2 protons into the intermembrane space.
2 Cyt c (reduced) + 4 H+N + 1/2 O2 → 2 Cyt c (oxidized) + 2 H+P + H2O
In summary:
- Complexes I and II transfer electrons to Q, reducing it to QH2.
- QH2 passes electrons to cytochrome c through complex III. (Both Q and cytochrome c are mobile electron carriers).
- Complex IV transfers electrons from reduced cytochrome c to O2.
- Electron flow through Complexes I, III, and IV is accompanied by proton flow from the matrix to the intermembrane space.
Figure 23.9 Summary of the movement of electrons through the ETC and the transfer of H+ from the matrix to the intermembrane space of mitochondria
CoQ acts as a collector of reducing equivalents
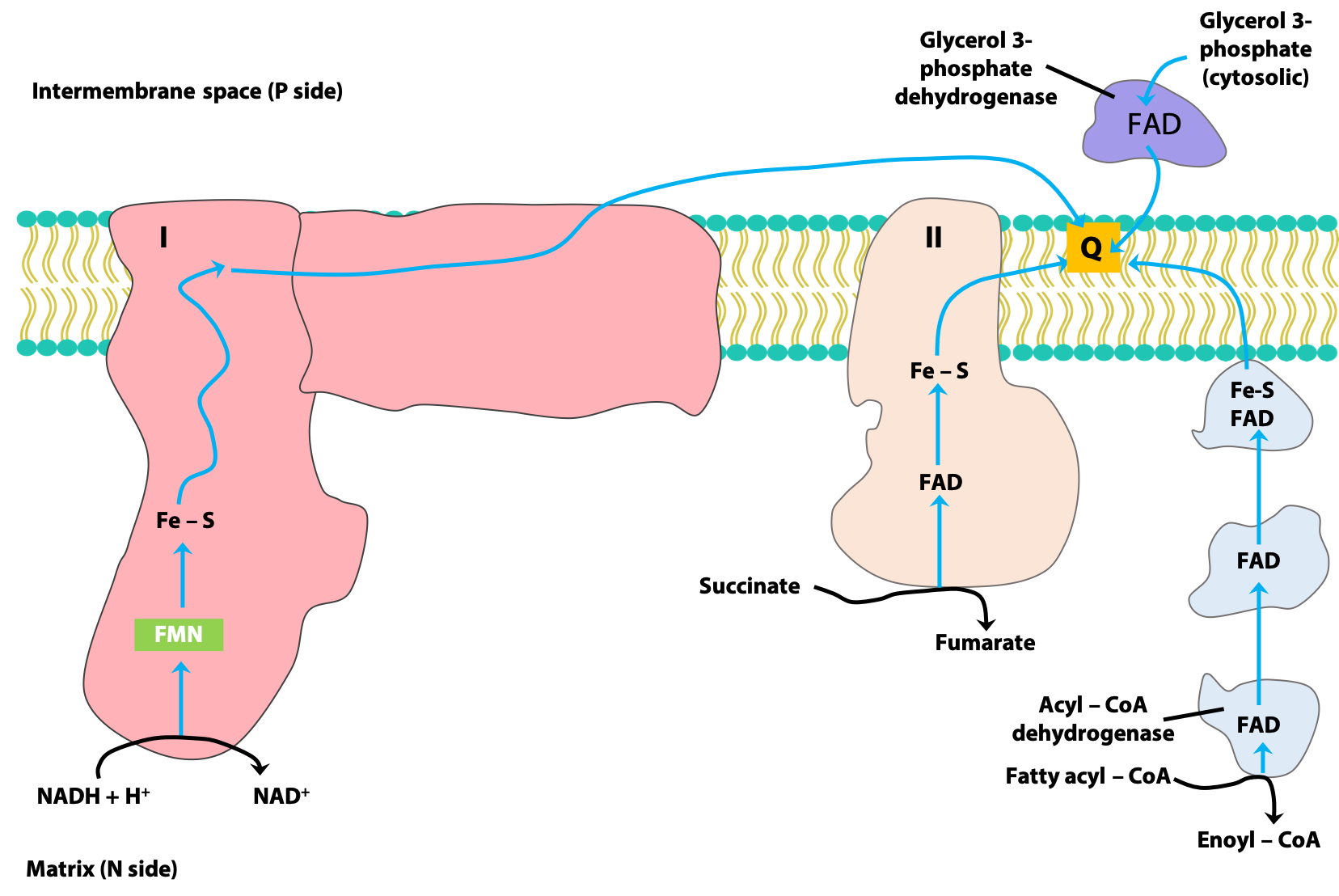
Coenzyme Q (CoQ) is, as we have just discussed, a lipid-soluble quinone. CoQ can easily diffuse within the lipid bilayer, and it acts as a “collection point” for reducing equivalents coming from several sources (Figure 23.10 and see Stryer, section 18.3.4). Here are some of the most important sources.
- NADH-derived reducing equivalents are transferred along the ETC to CoQ, via Complex I.
- Succinate dehydrogenase, the only membrane-bound enzyme of the citric acid cycle, is a component of Complex II. Reducing equivalents from FADH2 generated in this reaction are passed to CoQ, via the Fe-S protein components of Complex II.
- Recall that the first step of mitochondrial beta-oxidation is the oxidation of the fatty acid to an alkene, catalyzed by acyl-CoA dehydrogenase, and generating FADH2. These reducing equivalents are passed to CoQ, via a series of “electron-transferring flavoproteins”; Complex II is not involved.
- The enzyme glycerol-3-phosphate dehydrogenase of the glycerol 3-phosphate shuttle, which provides a route for the re-oxidation of cytoplasmic NADH, is associated with the inter-membrane space (i.e., cytoplasmic) surface of the IMM. Reducing equivalents from FADH2 generated by this enzyme are also passed to CoQ.
Determining the order of carriers in the electron transport chain
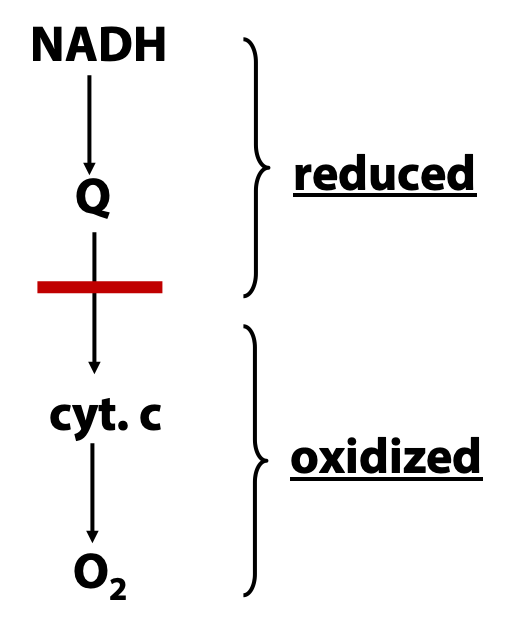
How do we know what the electron transport chain is made of, and how do we know the order in which electrons are carried? The composition of the electron transport chain has been determined by careful separation, purification, and identification of the various small-molecule and protein components (as described above) and then by reconstitution of the catalytic activities in vitro. But how can we figure out the order in which electrons are passed through the chain? To do this, we need to study the process as it occurs in the intact mitochondrion. The basic idea is one that we have already mentioned for other metabolic pathways: add an inhibitor, block the pathway, and see which intermediates accumulate (Figure 23.11). “Accumulating”, in the context of the electron transport chain, means “building up in the reduced state”. Why? Because when we block the chain somewhere, above the block, electrons keep pouring in from NADH , while below the block , they keep pouring out to oxygen, .
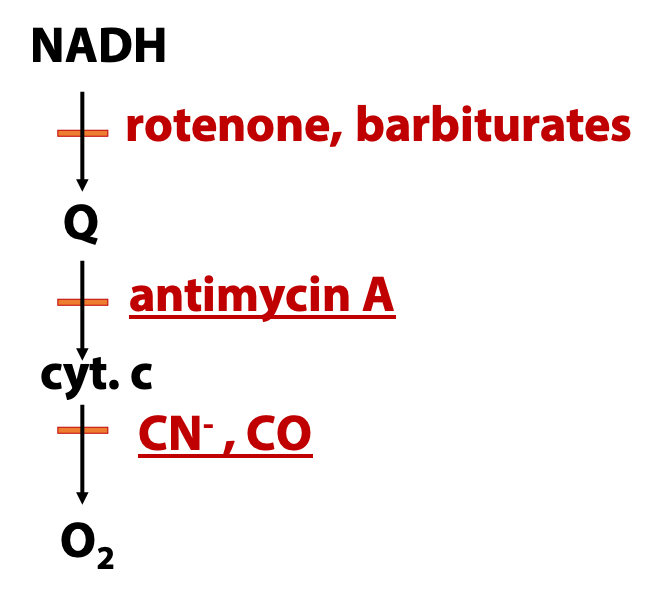
In order to do this with the electron transport chain, we need
(a) inhibitors and (b) a way of measuring the redox state of the carriers in the intact mitochondrion:
(a) Many respiratory inhibitors are known, as indicad in Figure 23.12, and they act at different points in the chain.
(b) Many of the carriers, such as coenzyme Q and cytochrome c, have distinctive optical chromophores.
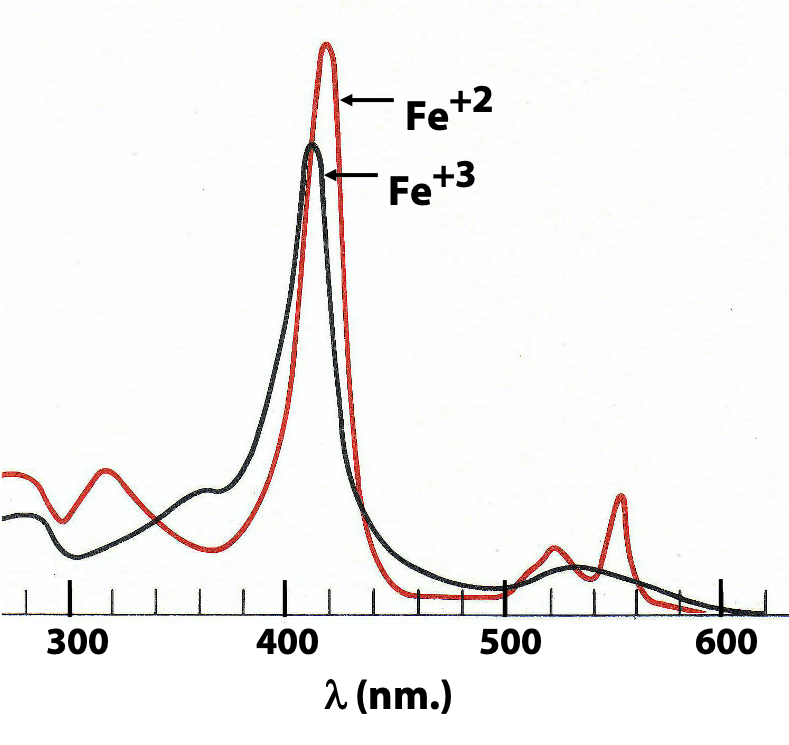
Figure 23.13 shows the optical spectra of cytochrome c in its reduced (ferrous) and oxidized (ferric) states, as measured with a sample of the pure protein. Notice that the big peak around 410 nm (called the “Soret band” after the French scientist who first studied it) shifts, while the peaks around 550 nm (the “alpha” and “beta” bands) in the reduced enzyme merge into one broad peak in the oxidized enzyme. We can use a spectrophotometer to watch these shifts happening to tells us the extent to which each of these carriers is oxidized or reduced. And then we replicate that under various conditions, with and without oxygen, with and without inhibitors, and so on.
The energy available through the oxidation of NADH and FADH2 is conserved in the form of a proton gradient
For each pair of electrons transferred from NADH to O2, four protons are pumped by complex I, four by complex III and two by complex IV for a total of 10 protons. Electrons from FADH2 bypass complex I and therefore a total number of 6 protons are pumped per each FADH2 oxidized. This creates a proton gradient across the inner mitochondrial membrane which acts as a temporary reservoir of much of the energy of electron transfer. The energy stored in the gradient is called as the proton motive force.