24 Oxidative Phosphorylation: Chemiosmotic Energy Transduction

Proton Motive Force has two components
- Chemical potential energy: Due to difference in concentration of H+
- 2.Electrical potential energy: Due to the separation of charges
Energetics of electron transfer
In actively respiring mitochondria:
electrical potential diff. (Δ Ψ ≈ 0.15 V)
chemical potential diff. (ΔpH ≈ 0.75 pH unit)
Free energy change for the transfer of 1 mole of H+ across the inner mitochondrial membrane:
Δ G = RT ln(C2/C1) + ZF Δ Ψ
= 2.3 RT Δ pH + F Δ Ψ
≈ 5 + 15 = 20 kJ mol-1
C2 & C1: concentration of H+ outside and inside the IMM
Z: absolute value of the electrical charge of H+ (1)
Δ Ψ: transmembrane difference in electrical potential
Total # of H+ pumped per mole of NADH oxidized through the ETC = 10
Total energy conserved in the proton gradient per NADH oxidized = 200 kJ
We can see that 200 kJ out of the 220kJ available from NADH oxidation is conserved in the proton gradient! The number of ions that need to be transported are small, but the energetic consequences are big. (The same is true in the case of neurotransmission, where small numbers of K+ and Cl– ions flow across gated ion channels in nerve cell membranes, resulting in large electrical impulses.)
How is this concentration of protons transformed into ATP?
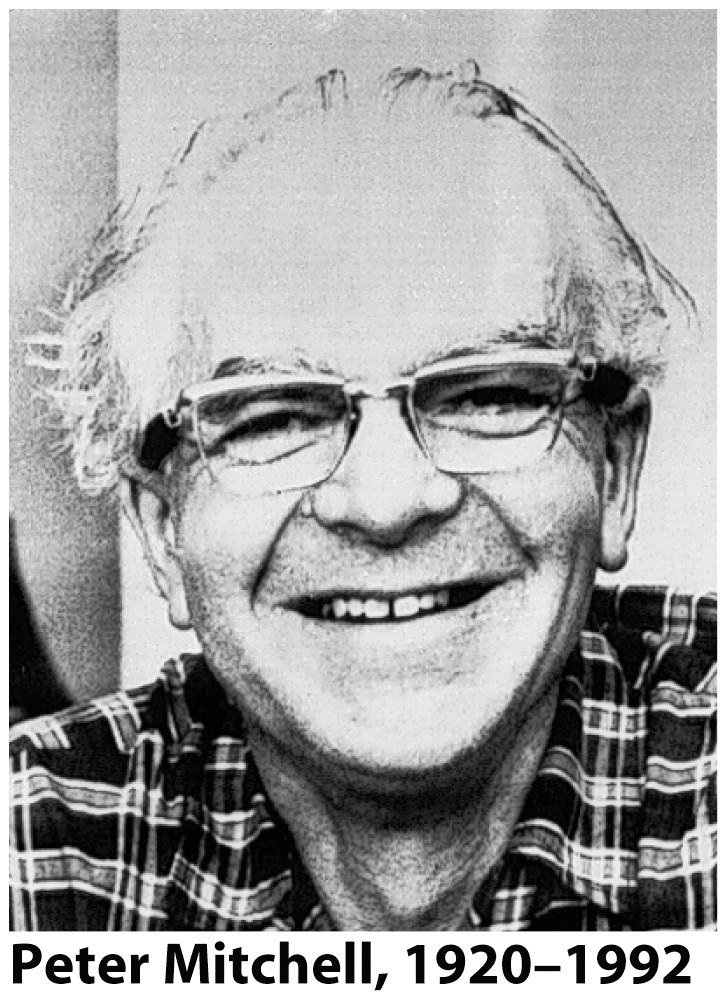
By about 1960, scientists knew that mitochondria generated ATP using the free energy liberated by oxidation of reduced cofactors by the electron transport chain. However, it was very hard to see how this system worked. Why? On the one hand, we have the electron transport chain, which involves redox reactions, not chemical bond formation. On the other hand, we have ATP synthesis, which involves forming a covalent chemical bond and has nothing to do with redox chemistry. This was until Peter Mitchell (Figure 24.2) proposed the chemiosmotic hypothesis (now the chemiosmotic theory) to explain the process.
The “chemiosmotic hypothesis” not only explained mitochondrial ATP generation but also turned out to explain lots of other important processes (like photosynthesis and bacterial motility and many membrane transport phenomena). Mitchell saw that the connection between electron transport and ATP synthesis was not mediated by any mysterious chemical messenger (the old “chemical-coupling” idea), but depended on generation of a chemical gradient across the membrane. And he realized the chemical had to be the one associated with redox phenomena – the proton, because most redox reactions (electron transfers) are accompanied by proton transfers: i.e., NAD+ + 2 e– + H+ → NADH
The Chemiosmotic Theory
- The free energy liberated by the redox reactions is used by the ETC to pump protons, moving H+ from matrix to inter-membrane space.
- Energy is stored as an electrochemical gradient (Proton motive force; by analogy to an electromotive force in an electric circuit)
- As protons flow back into the mitochondrial matrix down its concentration gradient, the energy of the electrochemical gradient is released and is used for the generation of ATP by the ATP synthase enzyme.
Mitchell thought of the mitochondrion as being like a battery (i.e., the electron transport chain) which charges up a capacitor (i.e., the inner membrane). But instead of running on a circuit of electrons, as a battery does, the system runs on a proton circuit. The chemiosmotic theory readily explains the obligatory coupling seen between electron transfer and ATP synthesis: neither reaction occurs without the other.

Mitchell’s idea also neatly explained another aspect of ATP synthesis in mitochondria: coupling. This could be easily demonstrated through the monitoring of oxygen consumption by mitochondria suspended in a buffered medium in an oxygen electrode apparatus (Figure 24.3, black line). Samples are removed at intervals to measure the presence of ATP (Figure 24.3, red line). Figure 24.3 also shows that inhibitors can block electron transport (CN–) can also block ATP synthesis. This is expected because it is substrate oxidation that provides the energy for ATP synthesis.

But here’s the twist: if we stop ATP production, electron transport stops – reoxidation of NADH stops! This is demonstrated in isolated mitochondria by providing oxidizable substrate (succinate) and O2 but not ADP (Figure 24.4). Under these conditions both oxygen consumption and ATP synthesis do not occur. The use of inhibitors of ATP synthase (oligomycin or venturicidin) will also bring both electron transport and ATP synthesis to a halt. Why should that be? The chemiosmotic theory clearly explains the dependence of electron transfer on ATP synthesis. It is the return flow of protons through the ATP synthase that powers ATP synthesis and discharges the proton gradient. If return flow of protons is blocked, the proton motive force quickly becomes so large that the electron transport cannot overcome it. Proton translocation is an obligatory part of the catalytic cycle of the electron transport chain complexes; therefore, if they cannot pump protons, they can no longer perform electron transport.
Uncouplers
If we disrupt the integrity of the inner mitochondrial membrane, the proton gradient will be eliminated (i.e., protons can freely move across the inner mitochondrial membrane); electron transport continues; ATP synthesis stops. The system has become “uncoupled”.
Chemical uncouplers
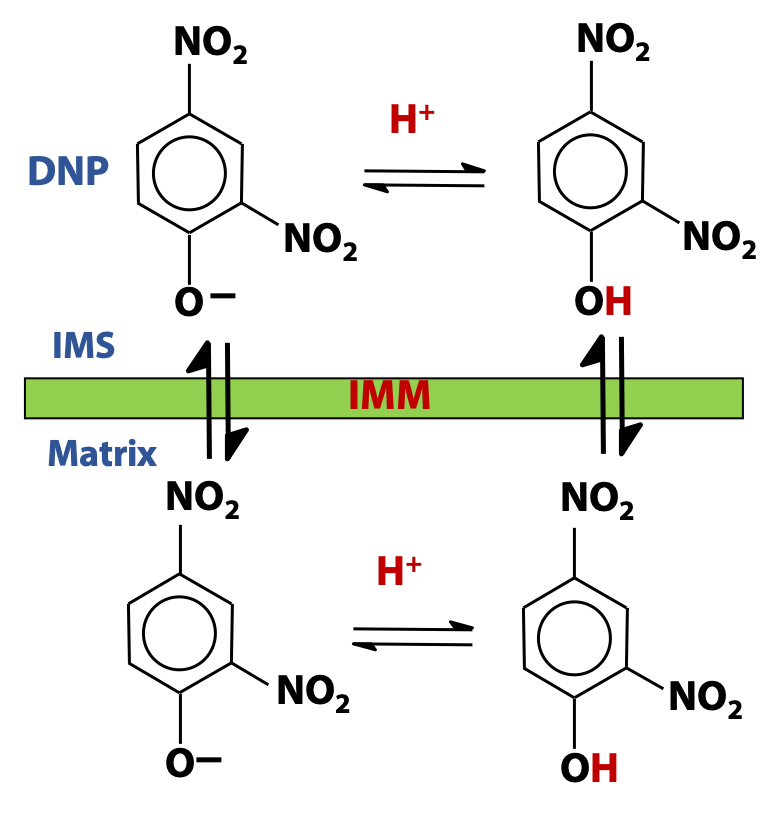
There are certain chemicals that have the same effect. Dinitrophenol (DNP) is the classic example of such a “chemical uncoupler”. When we add DNP to mitochondria, ATP production ceases, but electron transport continues (see Figure 24.4). It’s as though we had punched holes in the membrane. DNP is a fairly strong weak acid (pKa = 4), so the deprotonated (anion) form of DNP predominates, at neutral pH. The anion can pick up a proton, and the protonated neutral form can cross the inner mitochondrial membrane quite freely, since it is hydrophobic (Figure 24.5). The anion (conjugate base) form of DNP can also cross the membrane; its negative charge is delocalized over the aromatic ring and the structure retains considerable hydrophobic character. DNP and other uncouplers act as proton ionophores (ion carriers). DNP transports protons across the membrane – protons that are normally unable to cross, except by flowing through the ATP synthase. In the presence of DNP, the proton motive force collapses and the synthesis of ATP stops.