20 Fatty Acid Beta Oxidation
You can see from the numbers on your left that fat is the most concentrated store of metabolic energy. That’s why the First Nations peoples made pemmican: mixing a bit of meat (protein source) with lots of fat to make a foodstuff that you could carry on a long journey and would sustain you for a long time. It’s also how birds store enough energy to complete long migrations with little food.
Why is fat the most concentrated store of energy, per gram?
There are two reasons:
It is chemically very reduced – most of the carbon atoms are CH2. As a result, it will release the maximum amount of free energy when it is oxidized all the way to CO2. (In contrast, most of the carbon atoms in sugars are CH2O, so they are already partially oxidized.)
Fat is so hydrophobic that it can be stored nearly water-free. Sugars or proteins usually remain somewhat hydrated, even when you try to desiccate them, and water is just dead weight, as far as energy storage goes.
Nature has given us a series of energy reservoirs. As we burn through them (say, during a period of fasting) we move from readily-available but limited capacity reservoirs to much larger stores of energy that, however, are more difficult to mobilize. In animal metabolism, the order is (see right):
- ATP first
- Free glucose
- Stored glucose (glycogen)
- Fat.
There are lots of stored energy in protein, too, but this is mobilized very cautiously, because protein is too important, functionally. Fat isn’t as much important, although it does help keep you warm on a cold night.
Fatty acids are (mainly) found in TAG fat
Before fats (and we will only discuss TAGs) can be catabolized by beta oxidation, the ester bonds of the TAGs must be hydrolyzed to give the free fatty acids plus glycerol. Free fatty acids are only present at very low levels in cells; they are converted into other metabolites as quickly as they are made. When fatty acids are needed for energy metabolism, fats are broken down by lipases, enzymes that catalyze fat hydrolysis. The free fatty acids go on to oxidation. The glycerol is just a little bit of the mass and available free energy of the fat. It is converted into a glycolytic intermediate for catabolism. Under different metabolic conditions, such as postprandial satiety, fats are synthesized from fatty acids and glycerol, for storage.
Stages of Catabolism
The complete oxidation of fatty acids into CO2 and H2O is accomplished in three stages (Figure 20.1).
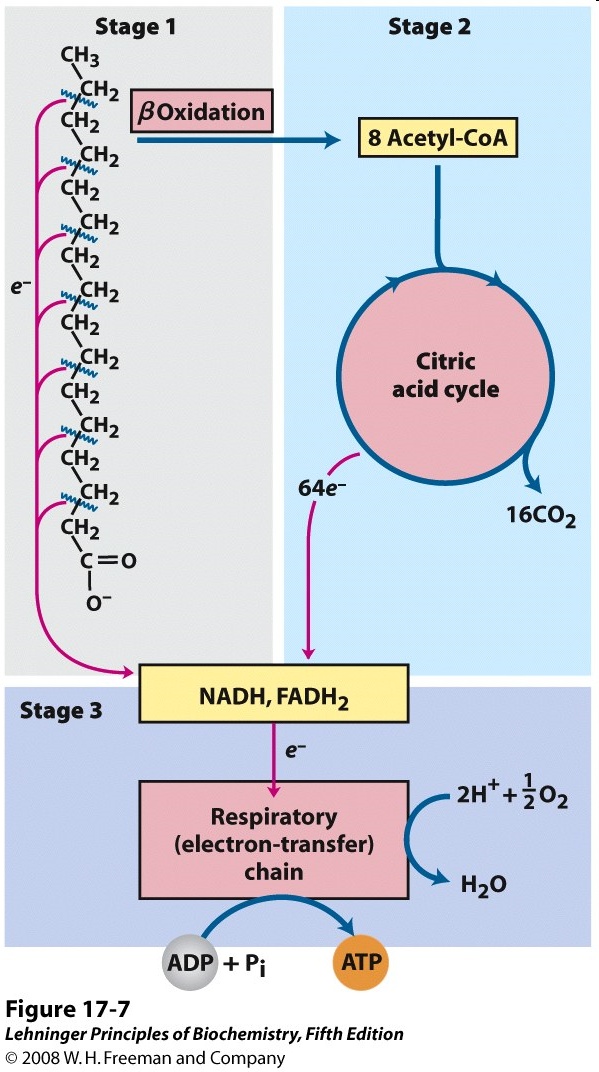
Stage 1: Beta oxidation (Fatty acid oxidation):
Two types of product are formed.
- The carbon atoms are converted to acetyl CoA, which is fed into the citric acid cycle.
- The other products are reduced cofactors.
Stage 2: The citric acid cycle
Stage 3: The electron transport chain
The oxidation of -CH2 carbons to CO2 requires oxidizing agents. The enzymes in fatty acid oxidation – and, indeed, in almost all metabolic processes – use just a very few oxidizing agents, enzyme cofactors such as NAD+ and FAD. As they oxidize fatty acids, these cofactors become reduced. Pretty soon, catabolism would grind to a halt, because the pools of NAD+ and FAD would become completely reduced, so no oxidizing agents would be available. But that doesn’t happen, usually, because molecular oxygen (O2) is used to re-oxidize the reduced cofactors. That’s called the electron transport chain, because it moves electrons (reducing equivalents) from the cofactors to oxygen. This is stage 3 of catabolism.
Elucidation of β oxidation (Franz Knoop; 1904)

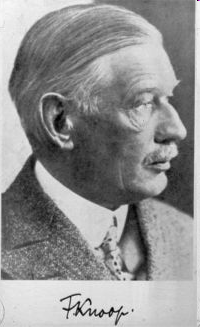
Today, we could figure out fatty acid oxidation easily, by using radiolabeled fatty acids and examining the products of their metabolism. But there were no radioisotopes available in 1904. So Franz Knoop (Figure 20.2) labeled fatty acids chemically, by tagging the terminal (-CH3) carbon atom with a phenyl group (Figure 20.3). Knoop fed these -phenyl fatty acids to dogs. After that, he collected their urine and isolated aromatic products by extracting them with an organic solvent. When the original fatty acid had an even number of carbon atoms in the chain, the product he got was phenylacetic acid (phenyl-labeled acetic acid), and when the original fatty acid had an odd number of carbon atoms, he got benzoic acid (phenyl-labeled formic acid). So the parity (even or odd) of the fatty acid was not lost or scrambled by metabolism. Knoop realized that this meant that fatty acids were catabolized two carbons at a time. And this must mean that the oxidation process occurred at the second (-CH2) carbon atom, not at the first (-CH3) carbon atom. Alpha oxidation would remove one carbon atom at a time.
Fatty acids are prepared for catabolism by activating them to fatty acyl CoA

Free fatty acids don’t hang around in cells for very long. Once fatty acids reach muscle cells, they are prepared for catabolism by conversion to fatty acyl CoAs, which are thioesters of the carboxylic acid group with the thiol (-SH group) of Coenzyme A. It requires ATP energy to synthesize those esters (Figure 20.4).
Acyl CoA synthetase occurs in the outer mitochondrial membrane. (A synthetase is an enzyme that catalyzes a reaction where two small molecules are put together to synthesize a larger molecule with the help of ATP energy. This is as opposed to a synthase, which is an enzyme that put two small molecules together to form a larger molecule without the help of ATP energy).
Note: In this reaction, ATP is hydrolyzed to AMP + 2 Pi, hydrolyzing both phosphoanhydride linkages of ATP. This is equivalent to the hydrolysis of 2 moles of ATP to 2 ADP + 2Pi.
The acyl CoA synthetase reaction mechanism
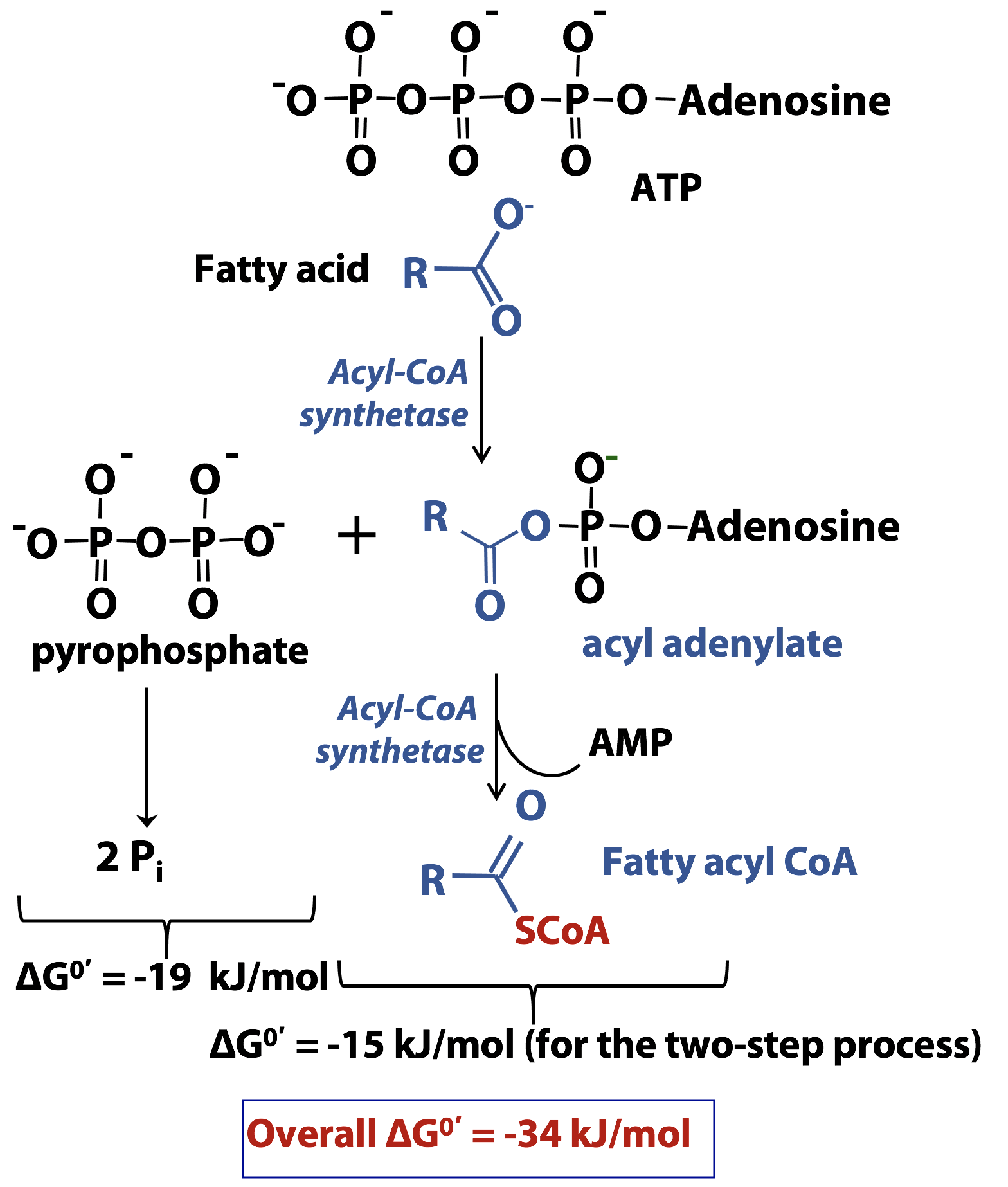
This is another example of how ATP is used in biosynthesis. The reaction mechanism is shown in Figure 20.5. The electron-rich oxygen atom of the acid is the nucleophile and the electron-deficient phosphorus atom of the ATP is the electrophile. The synthetase enzyme directs the nucleophile to the alpha phosphate , not the beta or gamma phosphate of ATP. So the products are pyrophosphate (PPi) and an acyl adenylate. The enzyme pyrophosphatase subsequently catalyzes the hydrolysis of pyrophosphate to give two moles of inorganic phosphate for every mole of ATP, helping to drive the formation of the acyl adenylate. Now, the unreactive carboxylic acid has been converted into a much more reactive acyl adenylate.
In the second part of the reaction catalyzed by acyl-CoA synthetase, the enzyme stabilizes the thiolate anion form of coenzyme A and directs it, as the nucleophile, to react with the acyl adenylate. AMP is the leaving group and the fatty acyl CoA thioester is formed.
While fatty acyl-CoA esters are formed in the cytosolic side of the outer mitochondrial membrane, β-oxidation of fatty acids occurs in the mitochondrial matrix. Fatty acyl-CoAs therefore must be transported into the mitochondria.
Compartmentalization is a major theme in eukaryotic biochemistry: specific tasks are performed in specific sub-cellular compartments. If molecules moved freely between compartments, there would be no point having compartments. But the membranes surrounding organelles act as barriers, much as the cell membrane does. As we see at the cell membrane, specific transporter proteins move molecules in or out of organelles.
The mitochondrion is the “powerhouse” of the eukaryotic cell. Most of the catabolic processes we are about to study take place in the mitochondrion (glycolysis is the notable exception). The mitochondrion probably started off as a free-living cell which was engulfed by a larger cell and began a symbiotic existence, remaining rather independent from its host cell: mitochondria still carry their own DNA and divide independently of the cell.
The mitochondrion is surrounded by a double membrane. The outer mitochondrial membrane contains aqueous channels that are freely permeable to molecules as large as some small proteins. Consequently, the inter-membrane space (the volume between the outer and inner membranes) is much like the cytosol, in terms of the small molecules that are present.
The inner mitochondrial membrane (IMM) is very different from the outer membrane. The IMM is highly involuted, giving it a very large surface area. The IMM is very rich in proteins – about 65% of the membrane’s mass is protein! And the lipid composition of the IMM is also unusual: it contains a lot of cardiolipin, a phospholipid that is hardly found anywhere else in the cell. Finally the IMM is highly impermeable to most solutes. Therefore, the mitochondrial matrix (i.e., the volume enclosed by the IMM) has a very different chemical composition from the bulk of the cytosol.
Transport of fatty acids into the mitochondria
Fatty acids (with >12 carbons) are transported across the mitochondrial membranes (outer and inner) in the form of esters with an alcohol called carnitine (fatty acyl carnitine esters) (Figure 20.6). Carnitine is a derivative of lysine. One form of carnitine acyl transferase acts at the outer mitochondrial membrane, converting the fatty acyl CoA thioester into a fatty acyl carnitine ester. A transport protein moves this ester across the inner mitochondrial membrane. Once it is inside the matrix, the fatty acyl carnitine ester is re-converted to a fatty acyl CoA thioester in a reaction catalyzed by another form of carnitine acyl transferase. The only fate of fatty acyl CoA molecules in the mitochondrion is beta-oxidation; they are committed to undergo catabolism, once they enter the mitochondrion.
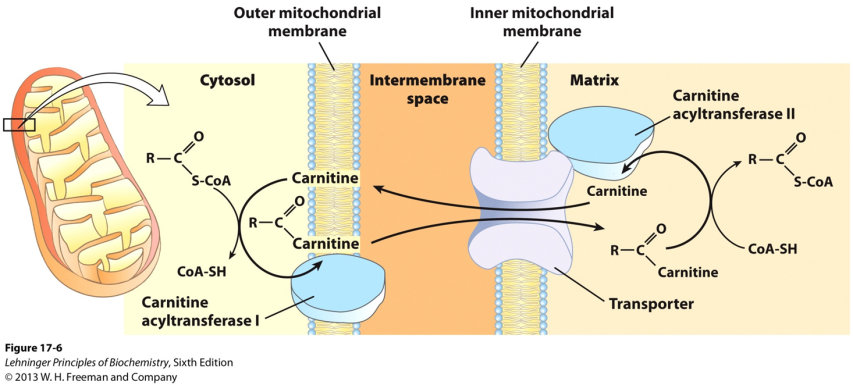
The Beta oxidation Pathway
Beta oxidation consists of four steps:
- Oxidation
- Hydration
- Oxidation
- Thiolysis
Each pass through beta oxidation removes one acetyl moiety in the form of acetyl CoA.
Step 1: Dehydrogenation by FAD forms a double bond between the α and the β carbons of fatty acyl CoA (Figure 20.7)
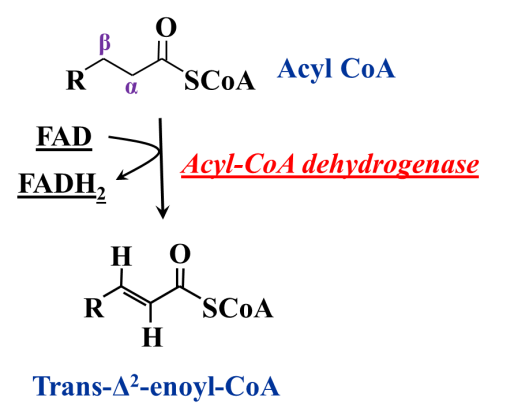
The first step of beta-oxidation is an … oxidation! It is hard to oxidize methylene (alkane-like) carbon atoms. In fact, it is almost impossible, unless the methylene group is activated (i.e., made more reactive by the influence of neighbouring functional groups). The bond between the alpha and beta carbons of the fatty acyl ester is somewhat activated, because the carbonyl oxygen atom of the ester is electron-withdrawing. But it is still hard to oxidize. The oxidant is FAD – not NAD+ – and the enzyme catalyzing this reaction is an oxidoreductase called acyl CoA dehydrogenase. The product is an alkene (a Δ2 alkene, because the position of unsaturation is between carbon atoms 2 and 3) and it is formed in the trans configuration (that is dictated by the enzyme). That makes it a trans “enoic” acid and so we call this product a trans-Δ2 – enoyl CoA.
Step 2: Water is added across the double bond to give an alcohol at the beta carbon (Figure 20.8)
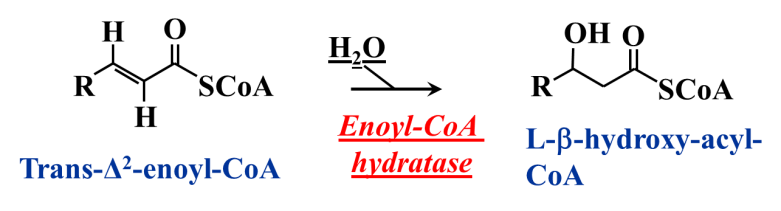
Next, the alkene is hydrated, i.e., the elements of water are added across the double bond, to give an alcohol at the beta carbon. This step makes the beta carbon chiral, but the enzyme dictates one specific stereoisomer formed, the L stereoisomer.
Step 3: Oxidation of the alcohol by NAD+ (Figure 20.9)
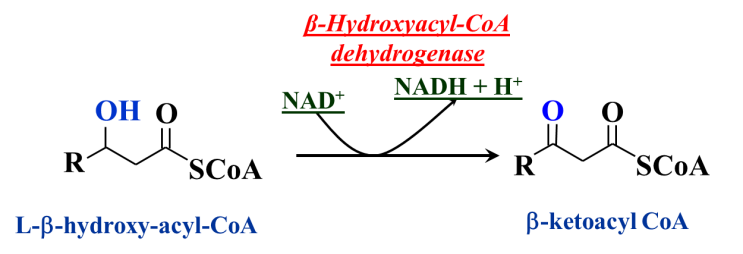
Alcohols are not very difficult to oxidize. The oxidant used in beta oxidation is NAD+ and the enzyme is hydroxyacyl CoA dehydrogenase. Note that this is a two-electron (2e– + 2H+) oxidation – an alcohol to a ketone. The reducing equivalents are transferred to NAD+, forming NADH + H+. Through these series of steps, the fatty acid has been oxidized, at the beta carbon, all the way from the methylene to the keto oxidation state. Consequently, the product of this last oxidation is a beta-ketoacyl CoA. Organic chemists appreciate that such beta-dicarbonyl (i.e., 1,3-dicarbonyl) compounds are especially reactive.
Step 4: β-keto acyl CoA reacts with coenzyme A, releasing its carboxyl-terminal two carbon fragment as acetyl CoA (Figure 20.10)
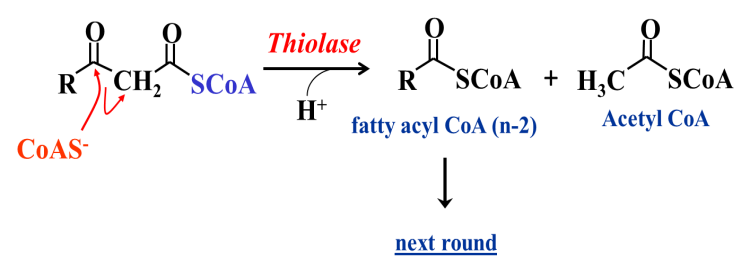
And we come to how the final step of beta oxidation goes. Firstly, coenzyme A binds to the enzyme thiolase – a basic group on the enzyme strips the proton from the thiol group of CoA, converting it to its ionized (thiolate anion) form. The thiolate anion (CoAS–) is a strong nucleophile, and the enzyme steers the anion so that it attacks the electrophilic (carbonyl) beta carbon atom. A C-S bonded tetrahedral intermediate results (oxyanion-not shown here). This intermediate also has a second carbonyl group, namely the C1 carbonyl. That oxygen atom “pulls” the electrons, which flow towards it (as shown by the arrows), breaking the bond between the alpha and beta carbons. Acetyl CoA is released as an anion, which (following protonation by the enzyme) gives acetyl CoA as a product, as indicated. The other product is simply a fatty acyl CoA, shortened by two carbon atoms. The reaction can be viewed as the thiol analogue of a hydrolysis, i.e., a “thiolysis”; hence, the common name of the enzyme catalyzing this reaction: thiolase. Note that the C-C bond cleavage is made possible by the beta-dicarbonyl functionality of the substrate.
How many rounds of beta oxidation are required to convert palmitoyl CoA (C16:0) into eight Acetyl CoA molecules?
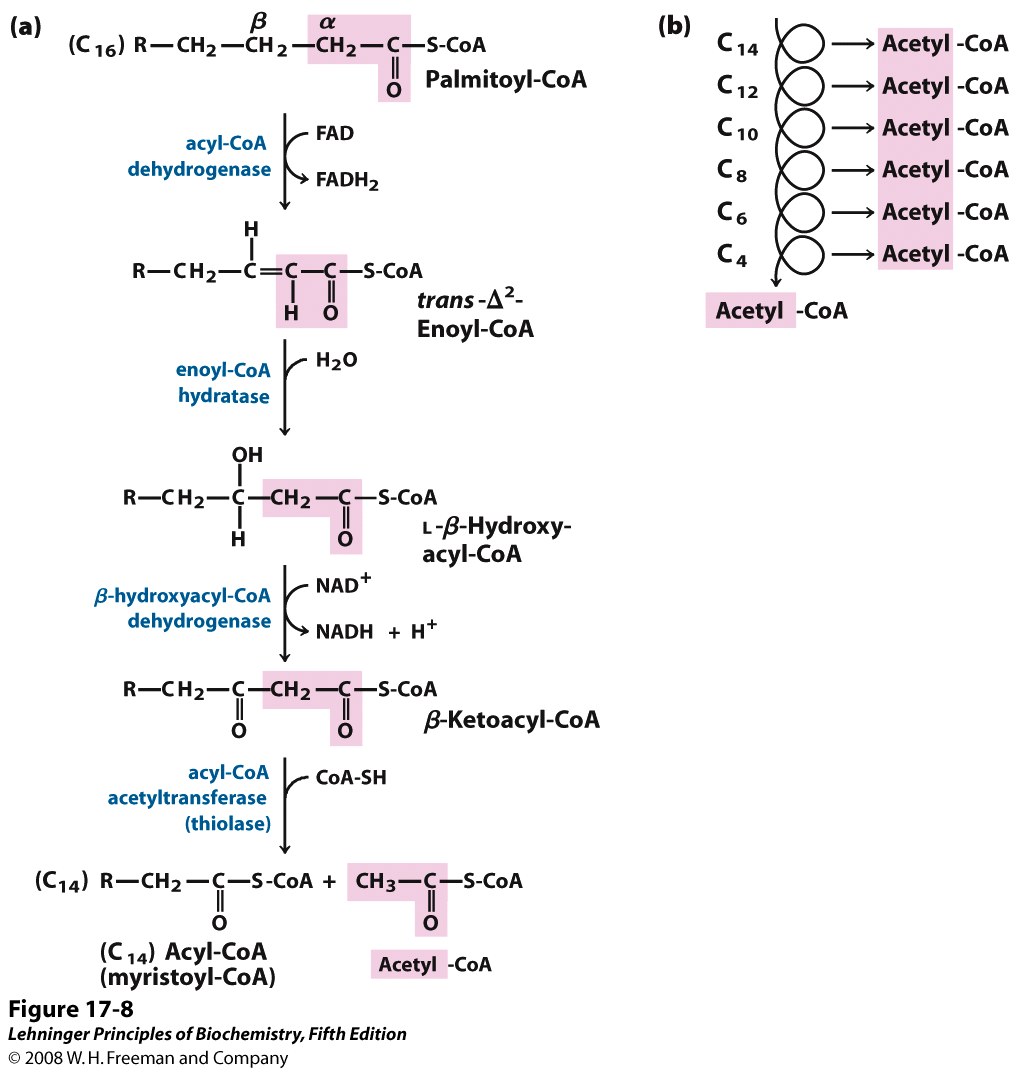
Suppose you have a ten-foot-long plank and you want to cut it down into two-foot-long sections. Of course, a ten-foot-long plank will give five two-foot sections. Then how many cuts must we make? The careless carpenter says “five”, but the thoughtful carpenter says “four!”
Similarity, the oxidation of palmitic acid into eight molecules of acetyl CoA requires seven passes through the beta oxidation sequence (Figure 20.11).
-1 FADH2 is formed in each turn (7 FADH2 in total)
-1 NADH is formed in each turn (7 NADH in total)
FADH2 and NADH will be oxidized via the electron transport chain. Acetyl CoA will enter the citric acid cycle and be completely oxidized to CO2.
Overall stoichiometry of beta oxidation (for palmitoyl CoA):
How are unsaturated fatty acids dealt with? What about fatty acids that have odd numbers of carbon atoms in the chain (they are uncommon, but they do exist)? All these are complex issues that the cell has to deal with. These topics will be covered in BIOC3560 and other courses.