21 Glycolysis
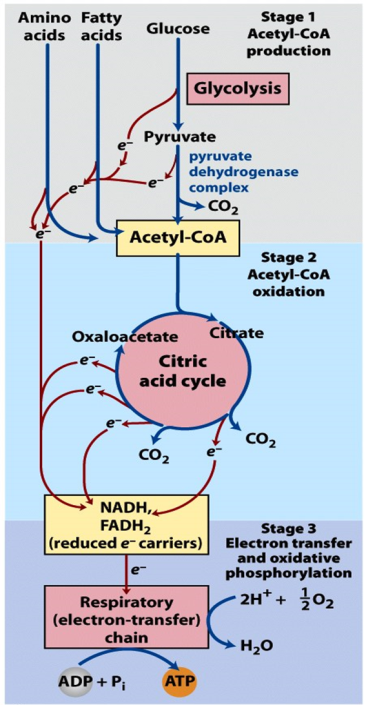
Complete oxidation of glucose to CO2 and H2O is accomplished in three stages (Figure 21.1).
Stage 1: Glycolysis + pyruvate dehydrogenase
Stage 2: TCA cycle
Stage 3: Electron transport chain
Glycolysis is the central pathway in carbohydrate metabolism and, as a result, in metabolism overall. Glucose is a common hexose sugar. It occurs as the monosaccharide (simple sugar), combined with fructose in the disaccharide sucrose (table sugar), and in polymeric forms such as glycogen, cellulose, and starch.
We will use the term glycolysis (from the Greek words for sugar + breakdown) for the sequence of reactions leading from glucose to the three-carbon compound pyruvic acid. There are many additional pathways that lead from other sugars to glucose (convergent catabolism), but we will not discuss them here.
Overview of carbohydrate metabolism
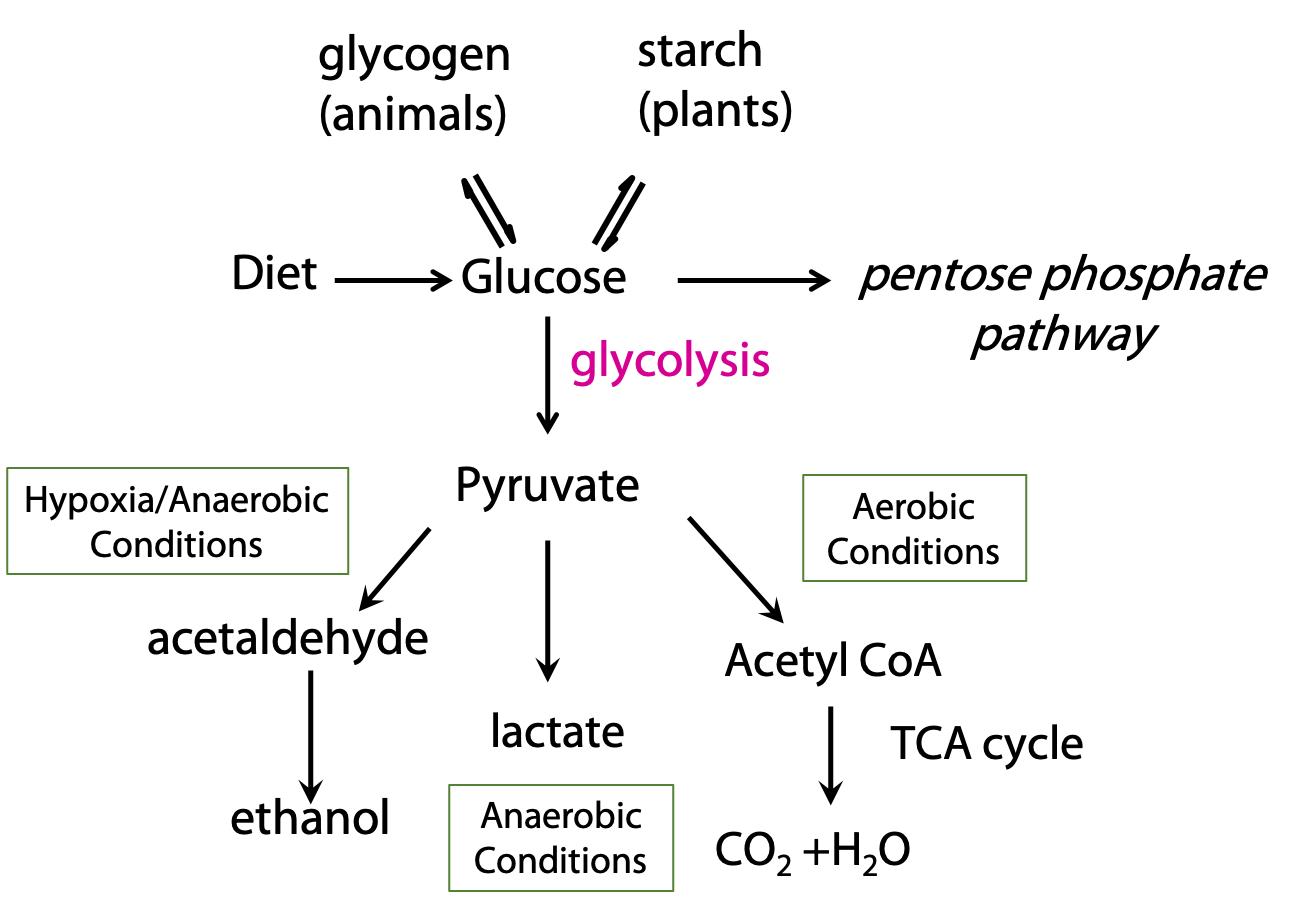
Before we look at glycolysis, let’s consider how it connects to other catabolic processes (Figure 21.2).
Pyruvate, the end product of glycolysis, can be oxidized completely to carbon dioxide, when oxygen is available. This occurs by the oxidative decarboxylation of pyruvate to acetyl CoA, which is then oxidized by the citric acid cycle. In the absence of oxygen, glycolysis can still be carried out; pyruvate is converted to ethanol. This is alcoholic fermentation, which is performed by some bacteria and yeast. In mammals, under oxygen-starved conditions, pyruvate is converted to lactate instead of acetate.
Transport of glucose into cells
Glucose is a highly polar molecule and cannot enter cells by passive diffusion across the membrane. Transporter proteins called GLUTs (GLUcose Transporters), catalyze glucose import into cells. One of many actions of the hormone insulin is to stimulate GLUT-mediated glucose uptake in skeletal muscle and adipose tissue. In diabetes, the body “starves in the midst of plenty”, because blood glucose is not taken up into cells adequately. Blood glucose can go sky-high, causing major problems in the blood and in multiple organs. Glucose is excreted in the urine.
Glucose is a critical source of metabolic energy for many organs – notably the brain. The brain consumes about100 grams of glucose daily. Red blood cells, renal medulla and sperm are some other tissues or cell types solely dependent on glycolysis for the provision of energy. Therefore, it is not surprising that multiple mechanisms exist to maintain blood glucose levels. The usual concentration of blood glucose in a fasting human is about 5 mM, making it one of the most abundant small molecules in the body. Glucose is absorbed from the diet or released into the blood from the breakdown of glycogen (a polymer of glucose) stored in the muscles and the liver. Glycolysis is the only pathway that can provide energy under anaerobic conditions; anaerobic microorganisms are completely dependent on glycolysis for energy generation.
Glycolysis occurs in the cytosol and consists of 10 different steps. It can be thought of as comprising two phases (see Stryer Fig. 16.3):
Preparatory: Import of glucose into the cell; phosphorylation; hexose sugar is split into two triose sugars; Pay-off: ATP generation phase. But this is really just a distinction made by textbook writers; it’s all one seamless process.
Step I: Phosphorylation of glucose
Glucose is transported into the cell, and it might also get transported out, but (usually) that does not happen, because glucose is rapidly phosphorylated. Once the sugar gets phosphorylated, it is negatively charged and becomes very polar, leading to be locked inside the cell. Every metabolite in the glycolytic pathway (until we get almost to the end of the pathway) is phosphorylated.
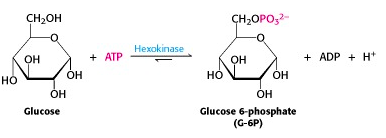
The ATP-dependent phosphorylation of glucose to give glucose-6-phosphate is catalyzed by hexokinase (Figure 21.3). This reaction is irreversible under intracellular conditions.
The human genome encodes four different hexokinases (I to IV), all of which catalyze the same reaction. Two or more enzymes that catalyze the same reaction, but are encoded by different genes are called isozymes. The isozymes present in hepatocytes (hexokinase IV/glucokinase), differ from the other three types in kinetic and regulatory properties with important physiological consequences.
Step II: Phosphohexose isomerisation
The phosphorylated glucose, an aldose sugar, is now isomerized to phosphorylated fructose, a ketose sugar, in a reaction catalyzed by phosphohexose isomerase. When we look at the pyranose and furanose ring structures of the sugars, this reaction seems mysterious. But the cyclic ring structures are in equilibrium with the open-chain forms. (In fact, the enzyme catalyzes the ring opening/closing). When you compare those structures, you can see that the reaction is just the interchange of the keto and hydroxyl functional groups. The mechanism of this reaction is similar to that of glycolysis enzyme #5, triose phosphate isomerase.
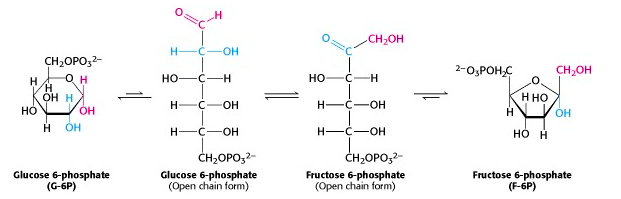
Mechanism of phosphohexose isomerase (for interest only)
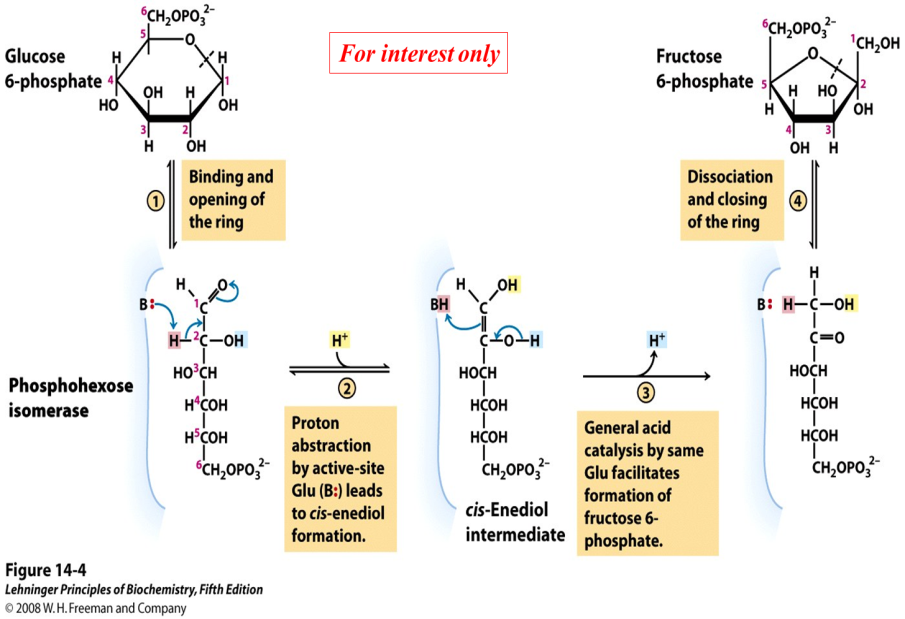
The reaction catalyzed by phosphohexose isomerase provides a good example of “general base catalysis”. First, the enzyme opens the pyranose ring to form the linear form of glucose 6-phosphate (Figure 21.5). Then a basic group on the enzyme (it’s the carboxylate anion form of a glutamate residue in the active site) abstracts a proton from C-2 of the aldose, as the C-1 aldehyde O picks up a proton from water. The product of these protonation/ deprotonation steps is an “ene-diol” (alkene + two hydroxyl groups) intermediate, bound to the enzyme. In the second step, a proton is lost from the C-2 hydroxyl group to the solution, as the C-1 carbon picks up a proton from the (protonated) glutamate residue on the enzyme. [Triose phosphate isomerase, enzyme #5 of glycolysis, functions through the same mechanism.]
Step III: The second phosphorylation
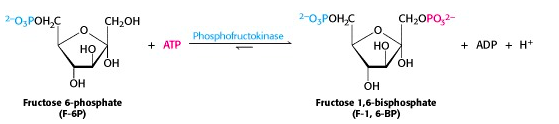
All the intermediates in glycolysis are phosphorylated. In the middle phase of glycolysis, the six-carbon sugar will be split into two three-carbon sugars, each of which will be phosphorylated. So we need to have two phosphates on the hexose, and here comes the second one (Figure 21.6). As usual, ATP is the source of the phosphate. Like the first phosphorylation reaction in step 1 of glycolysis, this reaction is also irreversible under intracellular conditions.
Why is the product called a “bisphosphate” rather than a “diphosphate”? Actually, it used to be called fructose-1,6-diphosphate. But we now use the term bisphosphate to describe a molecule that has two phosphates (esters) at different positions on the molecule, as opposed to ADP, for example, which has two phosphates joined to one another as an anhydride.
Step IV: The six-carbon fructose is split into two 3-carbon units
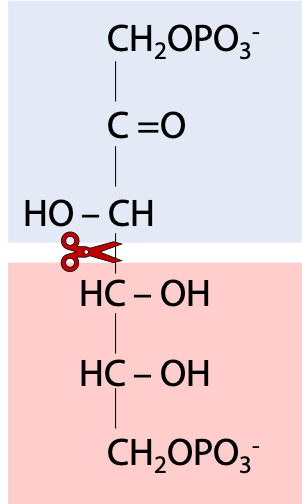
So, we are now roughly in the middle of the glycolysis pathway. Fructose-1,6-bisphosphate binds to the enzyme “aldolase”. (The name refers to the fact that the chemistry is (in the reverse direction to glycolysis) what an organic chemist calls an aldol condensation – an aldehyde plus an alcohol. One should be aware that there are several other enzymes called “aldolases” that catalyze similar chemical reactions but with different substrates). Aldolase is a lyase, an enzyme that splits one molecule into two (Figure 21.7).
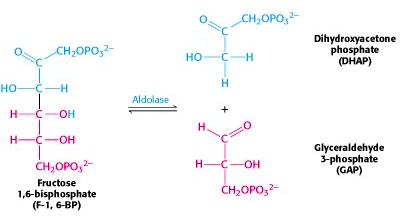
The six-carbon fructose is split into two 3-carbon units, dihydroxyacetone phosphate (DHAP) and glyceraldehyde-3-phosphate (G3P) (Figure 21.8). Note that these are phosphorylated derivatives of the two trioses, dihydroxyacetone and glyceraldehyde, which we talked about right at the start of our discussion of carbohydrate chemistry.
Step V: Interconversion of the triose phosphates
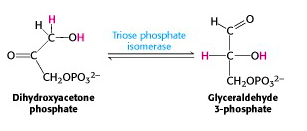
DHAP is immediately isomerized to G3P, and glycolysis continues from G3P only. The enzyme triose phosphate isomerase catalyzes the conversion of DHAP into G3P (Figure 21.9). Here, as in any symphony, we hear a reprise of an earlier theme: interconversion of an isomeric aldose/ ketose pair. That was how we converted phosphorylated glucose into phosphorylated fructose. Indeed, the mechanisms of triose phosphate isomerase and phosphohexose isomerase are essentially the same.
Note that, from this point onwards, two triose molecules must be catabolized for each molecule of glucose with which we started.
Step VI: Glyceraldehyde 3-phosphate dehydrogenase reaction: the energy key to glycolysis
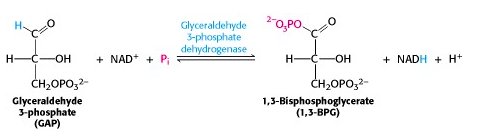
This reaction is the energy key to glycolysis. The two-electron oxidation of an aldehyde (e.g., glyceraldehyde) to a carboxylic acid (e.g., glyceric acid), using NAD+ as oxidant, is energetically highly favourable. In the enzyme-catalyzed process, G3P is bound to a cysteine residue on the enzyme G3P dehydrogenase and oxidized to an enzyme-bound thioester. The thioester is then attacked by inorganic phosphate, yielding the product 1,3-bis-phosphoglycerate (Figure 21.10).
This molecule is a mixed anhydride of phosphate and carboxylic acid, so it has a “high-energy bond”.
The G3P dehydrogenase step is an oxidation – it requires NAD+. To carry out glycolysis as an ongoing process, the resulting NADH must eventually be re-oxidized. We will return later to a discussion of how it is achieved.
VII: Phosphoryl transfer from 1,3-BPG to ADP: the first substrate level phosphorylation
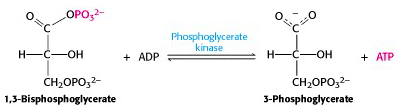
The free energy of hydrolysis of the anhydride bond is now recovered in the form of ATP, in a reaction catalyzed by phosphoglycerate kinase (enyzme #7) (Figure 21.11). Because the overall stoichiometry – two moles of 1,3-bisphosphoglycerate per mole of glucose, this reaction generates two ATP per glucose. The final steps of glycolysis capture the bond energy from the remaining phosphate ester bond in 3-phosphoglycerate.
Step VIII: Conversion of 3-phosphoglycerate to 2-phosphoglycerate
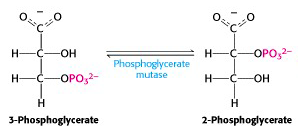
Glycolysis enzyme #8 is phosphoglycerate mutase (Figure 21.12). A “mutase” is a particular sub-class of isomerase: mutases catalyze reactions in which a functional group (in this case, it’s a phosphate) is shunted between different positions in a molecule (here, between the 2nd and 3rd position -OH groups of phosphoglycerate). The enzyme accomplishes this reaction by transferring a phosphate group from a side chain of the enzyme to the “vacant” -OH group of either 2- or 3-phosphoglycerate, producing the intermediate 2,3-bis-phospho-glycerate (a compound which turns out to have its own interesting physiological functions). After that, the enzyme accepts either one of the phosphate groups back. This exchange process interconverts substrate and product. As usual, consumption of the product (2-phosphoglycerate) in the next step of glycolysis drags this reaction towards completion, at steady-state, even though it is very close to thermodynamic equilibrium.
Step IX: Dehydration of 2-phosphoglycerate
The next enzyme, enolase – eliminates water to create a double bond. The systematic name for this enzyme is phosphopyruvate hydratase; it is named on the basis of the reverse reaction, the hydration of phospho-enol-pyruvate (PEP) (Figure 21.13).
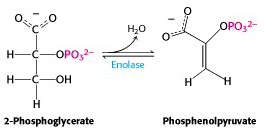
One must carefully distinguish between hydrolysis, in which water is added to a molecule and breaks it into two pieces (as in the hydrolysis of ATP to ADP + phosphate) and hydration, in which water is added to a molecule and the molecule remains intact (as in the hydration of PEP to give 2-phosphoglycerate). The reverse of a hydration is dehydration; in this case, dehydration of 2-phosphoglycerate gives PEP. The enzyme’s trivial name enolase refers to the product, PEP, which is an enol – an alkene/alcohol (the phosphorylated form of an alkene/alcohol).
Step X: Transfer of the phosphoryl group from PEP to ADP: The second substrate level phosphorylation
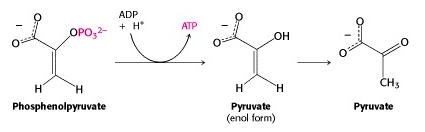
And now we come to the end of the glycolytic pathway. The last enzyme is pyruvate kinase. (Once again, it is named for the reverse reaction, the ATP-dependent phosphorylation of pyruvate.) Phosphoenolpyruvate (PEP) is a very high energy phosphorylated compound – look at Figure 16.1, and you will see it right at the top, well above ATP. So the free energy liberated by PEP hydrolysis is sufficient to drive ATP generation at this step. Pyruvate exists as an equilibrium mixture of keto (predominant) and enol (minor) tautomers. But PEP is “frozen” into the enol form, because its -OH group is phosphorylated. So its hydrolysis yields enolpyruvate; this quickly tautomerizes to ketopyruvate, pulling the pyruvate kinase-catalyzed reaction towards completion. With ketopyruvate, we declare that we have reached the “end of the glycolytic pathway”. Of course, this is a somewhat arbitrary declaration; pyruvate is further metabolized along several different pathways.
Maintaining Redox balance in glycolysis
Remember G3P dehydrogenase? It catalyzes an NAD+-dependent oxidation, namely, oxidation of the aldehyde group of glyceraldehyde (as glyceraldehyde-3-phosphate) to an acid. To carry out glycolysis as an ongoing process, the resulting NADH must eventually be re-oxidized. Now we must consider that question, in the context of various physiological conditions.
Anaerobic conditions (exercising muscles, RBC’s):
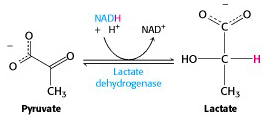
Under oxygen-deprived conditions, such as strenuous exercise, the supply of oxygen to the electron transport chain cannot keep up with demand for the reoxidation of NADH. The NAD+ needed by G3P dehydrogenase cannot be generated by mitochondrial metabolism, under these conditions. But glycolysis can continue: the muscles switch to using pyruvate itself to reoxidize NADH. Pyruvate is reduced to lactate (lactic acid when protonated), in a reaction catalyzed by lactate dehydrogenase (Figure 21.15).
Mature red blood cells (RBC’s) do not carry any organelles (e.g., they do not have mitochondria). Therefore, even under aerobic conditions they are unable to re-oxidize NADH via the electron transport chain. Glycolysis, under all conditions (aerobic and anaerobic), produces lactic acid in RBC’s. Anaerobic glycolysis is the only means of ATP generation available to RBC’s.
The Cori Cycle
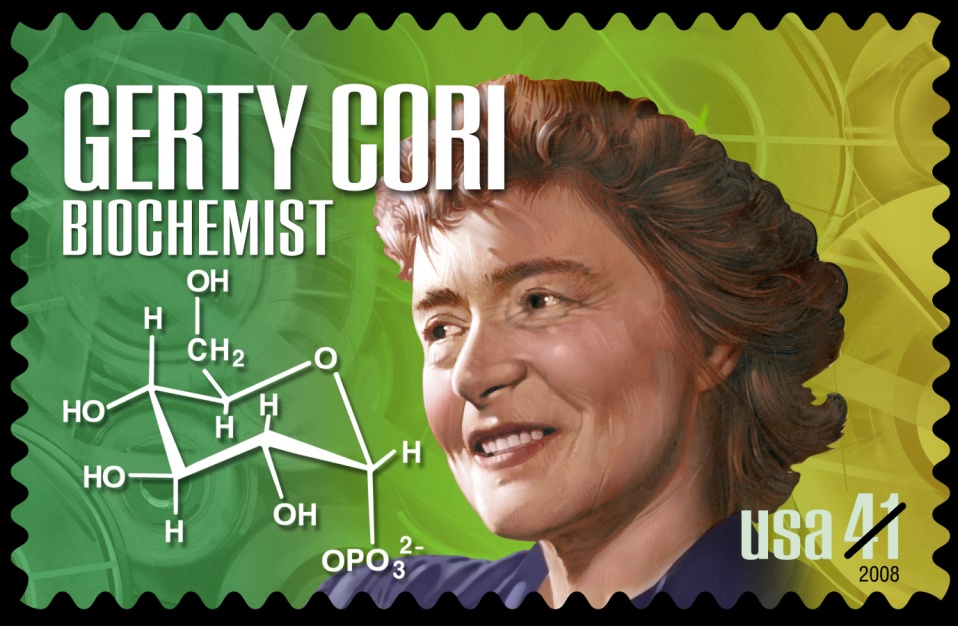
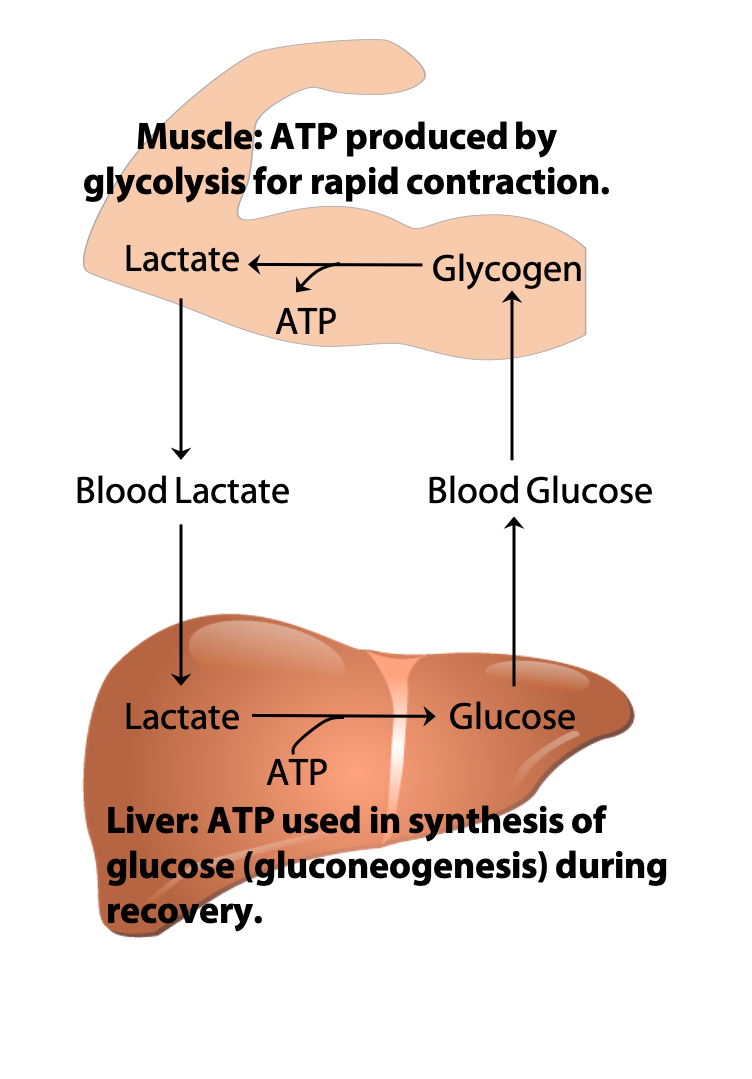
During recovery from strenuous exercise, some of the lactate produced in muscles is exported to the liver via the blood. The liver converts the lactate back to glucose, through the pathway known as gluconeogenesis, which will be discussed in BIOC*3560. The glucose is then returned to the muscles to replenish the amount of glycogen stored in muscles that were used during exercise. This exchange of metabolites between the organs is known as the “Cori cycle” (Figure 21.16) after its discoverers Carl & Gerty Cori (Figure 21.17) (Nobel Prize, 1947).
Yeast and other microorganisms convert pyruvate to ethanol and CO2 under anaerobic conditions: alcoholic fermentation
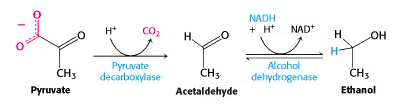
We now turn to the metabolic process that preoccupied the earliest biochemists – fermentation. Yeast has a different mechanism for carrying out glycolysis under anaerobic conditions: fermentation to ethanol. The enzyme pyruvate decarboxylase catalyzes loss of CO2 to give acetaldehyde. This carbon dioxide gas can be trapped in wine – it’s the bubbles in champagne – or released into fermenting dough, making bread rise. Animals do not have this enzyme, so we have to rely on yeast to make alcohol for us. Acetaldehyde is reduced to ethanol by the action of alcohol dehydrogenase, regenerating NAD+ for glycolysis. Animals also have alcohol dehydrogenase, which acts to carry out the reverse process: the NAD+-dependent oxidation of ethanol to acetaldehyde. This is how you remove alcohol from your system after drinking a glass of wine. (The resulting acetaldehyde is further oxidized to acetic acid.)
Aerobic conditions:
In animals, under aerobic conditions, the mitochondrial electron transport chain re-oxidizes NADH, with O2 as the ultimate oxidant, as we would see later. So, there is no problem – indeed, there is opportunity for additional ATP generation via oxidative phosphorylation. This is what is usually happening in your tissues, as you walk to school in the morning.
In this case, pyruvate is completely oxidized to carbon dioxide via the citric acid cycle. For this to happen however, pyruvate, which is formed in the cytosol, must first be moved into the mitochondrion. This is done by a special transporter protein. Once in the mitochondrion pyruvate is converted to acetyl CoA by the pyruvate dehydrogenase complex. Acetyl CoA can then enter the TCA cycle to get completely metabolized to CO2.
As noted earlier, the NADH generated by the G3P dehydrogenase reaction of glycolysis is re-oxidized via the electron transport chain under aerobic conditions. But there’s a problem – the glycolytic reactions are running in the cytosol and the electron transport chain is mitochondrial. In between lies the mitochondrial inner membrane, and NADH can’t pass through it (few metabolites can). So the solution to transport is the reducing equivalents*, rather than transporting the NADH itself. We’ll discuss two shuttle systems from different tissues below.
* electrons, possibly accompanied by protons (could be in the form of electrons alone (e), H atoms (e + H+) or hydride ions H–)
Malate –Aspartate shuttle (liver, kidney, heart)
This shuttle system (Figure 21.19) relies on redox interconversions of malate and oxaloacetate. In the cytosol, NADH reduces oxaloacetate to malate and then malate is transported into the mitochondrion by a specific transporter protein. In the mitochondrion, malate reduces NAD+ to NADH, regenerating oxaloacetate. Since there is no transporter that can move oxaloacetate back to the cytosol, oxaloacetate is converted to aspartate (through a process known as transamination), which then crosses the inner mitochondrial membrane through a transporter.
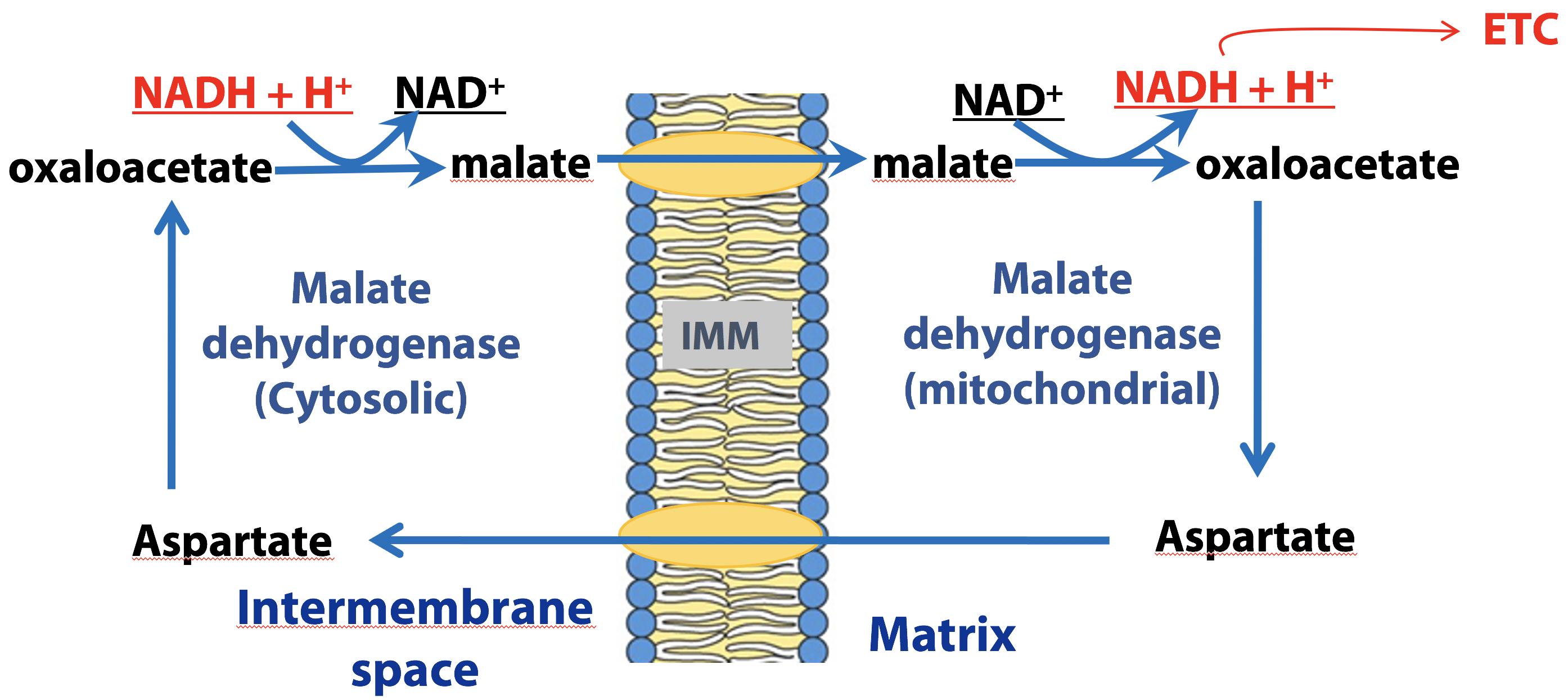
Once in the cytosol, oxaloacetate is regenerated from aspartate through the reversal of the transamination reaction that occurred in the mitochondrion. Both of the malate/oxaloacetate interconversions are catalyzed by malate dehydrogenase (either cytosolic or mitochondrial forms). This shuttle therefore, generates a mitochondrial NADH for each cytosolic NADH produced by glycolysis under aerobic conditions.
Glycerol 3-phosphate shuttle (esp. brain, muscles)
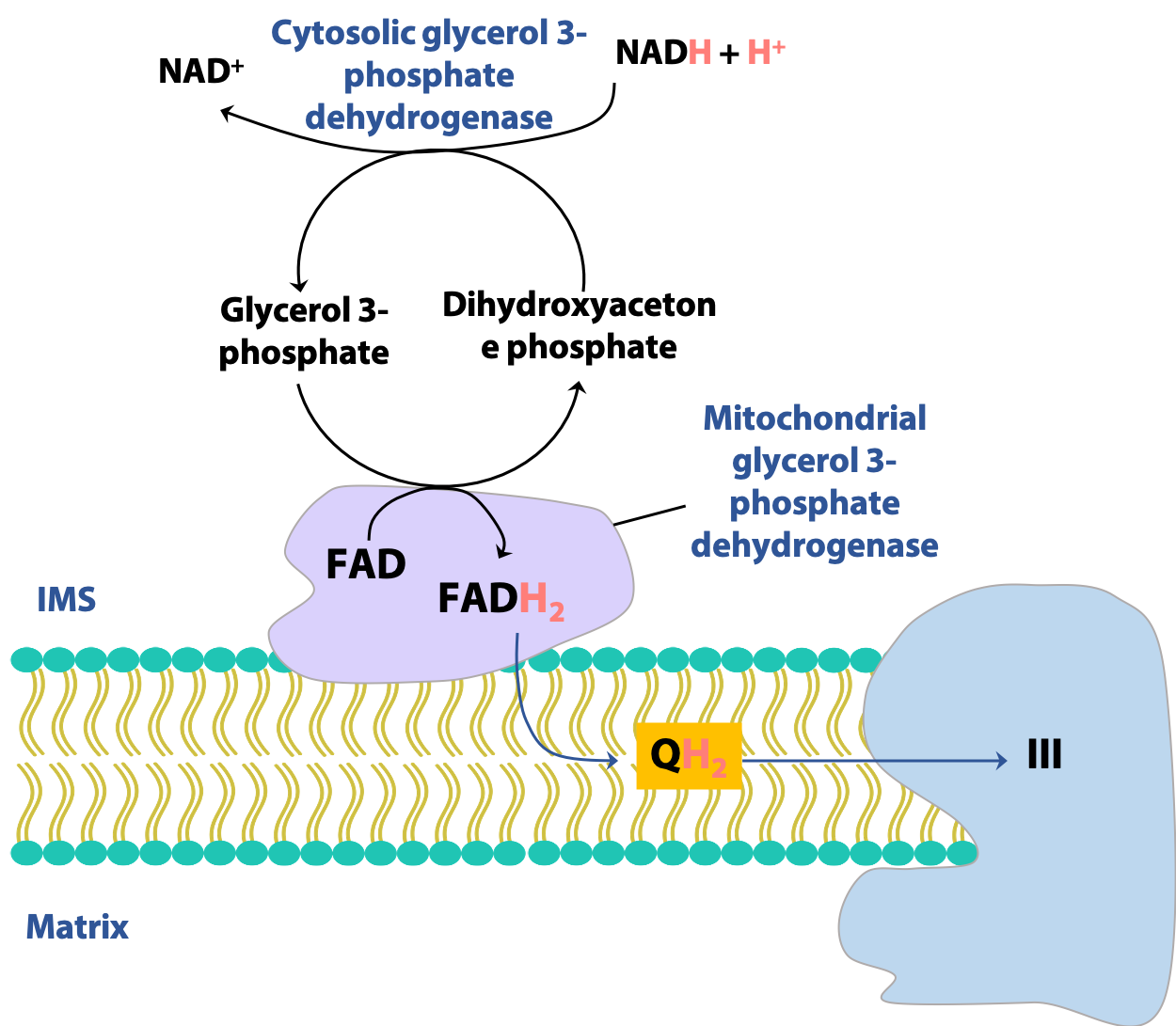
There are more than one way to transfer reducing equivalents between the cytosol and mitochondrion. In certain tissues, notably the muscles and the brain, another shuttle is active, the glycerol-3-phosphate shuttle (Figure 21.20). Here, the redox reaction is the interconversion of dihydroxyacetone phosphate (DHAP) and glycerol-3-phosphate, catalyzed by glycerol-3-phosphate dehydrogenase. The mitochondrial form of the enzyme uses FAD rather than NAD+ to oxidize glycerol-3-phosphate. Consequently, this shuttle generates a mitochondrial FADH2 for each cytosolic NADH, with consequences for the overall energy yield of glycolysis, as we will see next.
Note: Don’t confuse glycerol-3-phosphate (formed by the reduction of dihydroxyacetone phosphate) with the glycolytic intermediate glyceraldehyde-3-phosphate (formed by the isomerization of dihydroxyacetone phosphate).
Pyruvate dehydrogenase complex (PDH)
This enzyme occupies a key position in metabolism: it is the link between glycolysis and the citric acid cycle. In fact, in Krebs’ day, it was assumed that pyruvate itself was the starting point of the citric acid cycle. But that was before Lipmann discovered acetyl CoA and recognized its central role in metabolism. The pyruvate dehydrogenase complex (PDH) is the connection (Figure 21.21): it converts pyruvate (the end product of glycolysis) into acetyl CoA, the first metabolite of the citric acid cycle, condensing with OAA to make citrate.
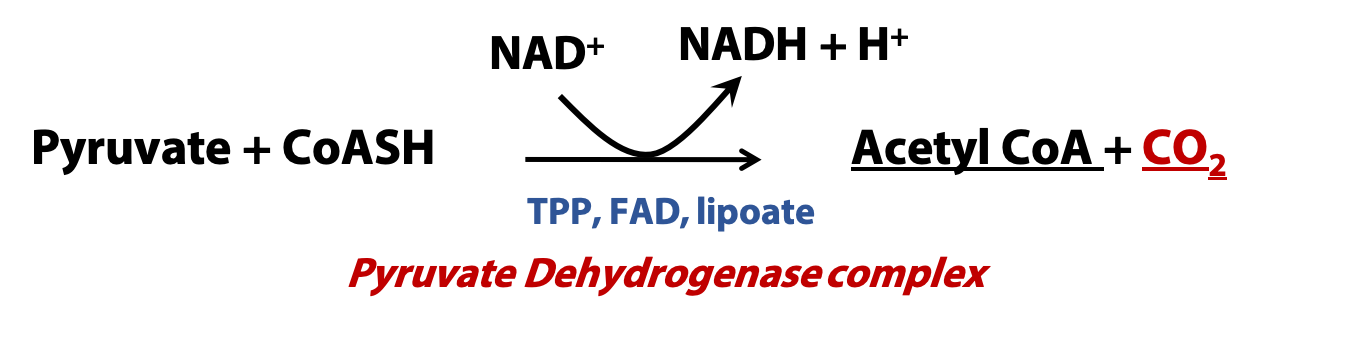
PDH occurs in the mitochondrial matrix and catalyzes an irreversible oxidative decarboxylation. (α-ketoglutarate dehydrogenase, in the citric acid cycle catalyzes the analogous reaction, but with α-ketoglutarate rather than pyruvate as the α-keto acid substrate). Indeed, PDH and α-ketoglutarate dehydrogenase, although are distinct enzymes, are closely related and have identical mechanisms.
Pyruvate dehydrogenase complex consists of three different enzymes and utilizes 5 different cofactors; four of which are derived from B vitamins.
(For interest only; not to be tested)
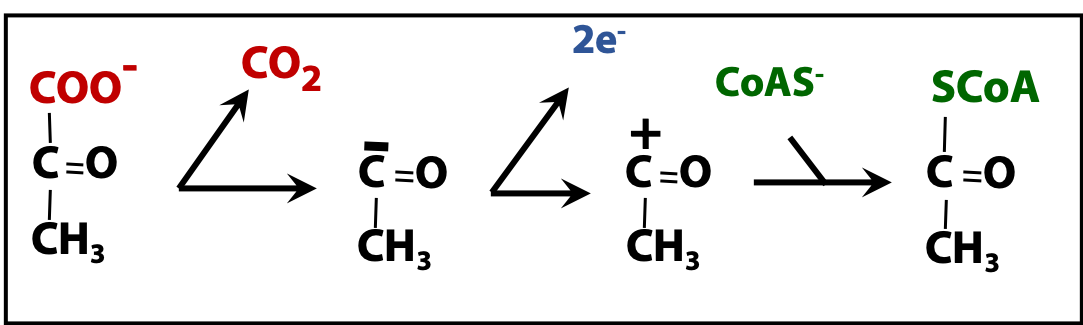
Here’s a brief look at the chemistry of this multi-step reaction (Figure 21.22). The decarboxylation (loss of CO2) from pyruvate yields acetaldehyde (HC(=O)CH3), in its deprotonated form¯C(=O)CH3. (Actually, this is an intermediate, covalently bound to a cofactor component of the PDH enzyme.) The next step is the NAD+-dependent oxidation of the acetaldehyde equivalent to a carboxylic acid equivalent, which is acetate. You can see this if you think of acetic acid (CH3COOH) losing ¯OH to give (formally) +C(=O)CH3. And this joins to the anion form of coenzyme A (CoAS¯) to give CoASAc.