8 Protein binding & Recognition: Chemical Basis of Enzyme Catalysis
Synopsis: The purpose of many proteins is to bind and recognize specific target molecules. Effective binding occurs through interaction very similar to the interactions that cause proteins to fold up. Specific binding results from cavities and regions on the protein surface which complement the target molecule
Enzymes catalyze a reaction on their bound target, speeding up reactions by a variety of catalytic effects, including proximity effect, orientation effect and chemical catalysis. Chemical catalysis lowers the activation energy Ea required for reaction by finding a better chemical pathway for reaction. This may be achieved by nucleophilic catalysis, electrophilic catalysis, general acid or general base catalysis or by transition state stabilization.
Protein binding and recognition of other molecules.
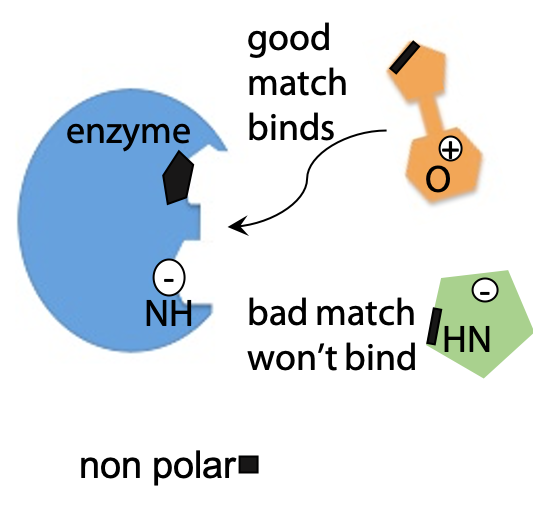
Proteins fold because one part of the polypeptide chain binds and recognizes another specific part of the same polypeptide. Exactly the same types of non-covalent interactions that cause a protein to fold can also be used to allow a protein molecule bind any other kind of molecule (Figure 8.1). Strong binding interactions require a complementary match between protein and bound molecule, so that binding is highly specific, and serves as a molecular recognition mechanism.
- Nonpolar patches on the protein surface bind by hydrophobic effect
- Complementary shapes maximize close contact – van der Waals effect
- Charged or H-bonding groups line up with complementary partners, e.g. Donor with acceptor, +ve with –ve.
Examples
Proteins that have quaternary structure bind other protein molecules of the same type to form multiprotein complexes.
Enzymes are proteins that bind and identify other molecules and catalyze a specific reaction.
Antibodies are proteins that bind and recognize foreign molecules, e.g. on invading bacteria or viruses, antibodies tag them for attack by other components of the immune system.
Proteins have very diverse shapes, making them ideal to form regions on their surface which match and complement a particular target molecule.
Each protein has a unique shape and can bind specific target molecules.
Binding and Bonding: These terms may seem interchangeable in everyday speech, but not in biochemistry. If a protein binds another molecule, the interaction is non-covalent and usually freely reversible. Covalent links between two parts of a molecule are referred to as bonding.
Example – binding and recognition of target peptides by peptidases of the chymotrypsin family (Figure 8.2):

Peptidase = generic name for enzymes that catalyze hydrolysis of peptide bonds. Chymotrypsin and a number of related enzymes share a common core structure responsible for binding the target peptide. A groove in the chymotrypsin molecule accommodates a target peptide chain, forms hydrogen bonds to its backbone CO and NH groups. This interaction is very similar to binding an extra β-sheet strand of its own. In the figure, the components of the enzyme are in red, and the components of the target molecule are in black.
A hydrophobic pocket in the chymotrypsin fits a side chain with an aromatic ring, e.g. Phe, Tyr or Trp. Binding of the target peptide is much tighter if this pocket is occupied by an appropriate amino acid. This binding positions the target peptide bond on the carboxylate side of the aromatic amino acid next to the catalytic component of chymotrypsin, which then proceeds to hydrolyze that bond.
The binding pocket is formed by amino acids Gly, Trp and Leu of the chymotrypsin molecule (Figure 8.2, shown in red), which have hydrophobic properties to match the target.
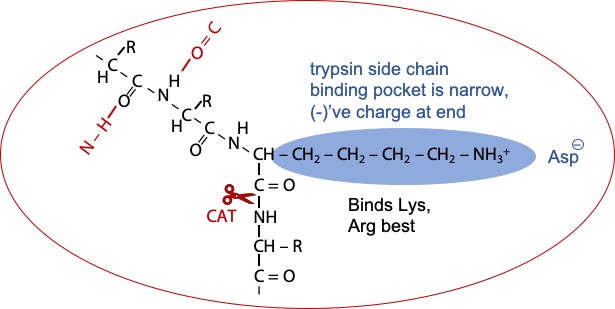
Trypsin is similar, but the pocket is narrower and the trypsin has a negatively charged aspartate in the back of the pocket. The target peptide now fits best in the binding pocket with an amino acid having a long side chain with a positive charged at the end (Figure 8.3). Hence, Lysine or Arginine are bound and recognized; and hydrolysis occurs on the next peptide bond.
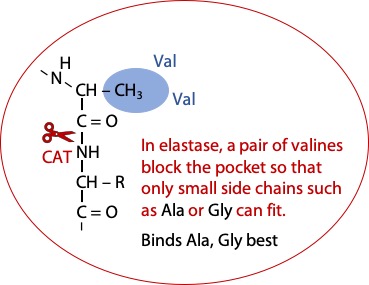
The enzyme Elastase is similar to chymotrypsin, except that part of the pocket is blocked off by a pair of bulky valines. The best fit for the target peptide is alanine or glycine (Figure 8.4).
Enzyme catalysis
Many proteins are enzymes, whose functions are to bind target molecules and catalyze a specific reaction. The suffix -ase at the end of a protein name indicates an enzyme, e.g. ribonuclease, an enzyme that breaks down RNA – ribonucleic acid by hydrolysis. Other example is peptidase, an enzyme that breaks peptide bonds by hydrolysis, etc.
The target molecule bound to and react on the surface of the enzyme is called the substrate of the enzyme.
Enzymes catalyze reactions that might otherwise occur spontaneously, but at a very slow rate. Enzymes are not magic – the reaction must be chemically feasible in the first place. Enzymes may speed up reactions anywhere from 106- to 1017-fold.
How is the enzyme so efficient when uncatalysed reactions are slow?
Uncatalysed reactions may be relatively slow because of the random nature of the
process.
Molecules must first collide*, and they must also be in the right orientation and possess a critical threshold energy, in order to react
*collisions are important for unimolecular reactions as well as bimolecular, since molecules can exchange energy through collisions.
From simple chemical kinetics
rate = p.Z.e-(Ea/RT)
Z is the collision frequency,
p is a probability factor, which has a lot to do with the relative orientation of the reacting molecules.
Ea is called the activation energy, the minimum energy a molecule must possess to initiate the reaction. e-(Ea/RT) is the fraction of molecules at temperature T (in degrees Kelvin) which possess energy Ea. This value tends to zero as Ea increases (Ea is always positive), so Ea should be as low as possible.
We can speed up a reaction if we can increase p or Z, or decrease Ea.
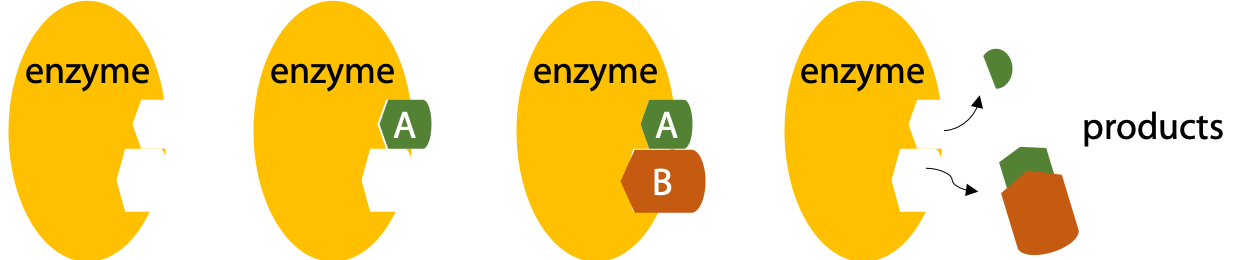
Enzymes bind their substrates to reduce the randomness of collision (Figure 8.5)
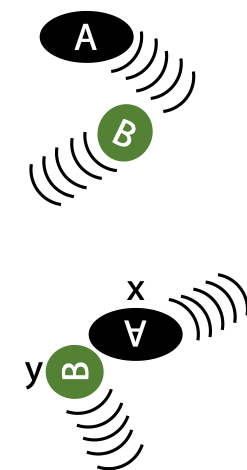
Proximity effect: enzymes bind their substrates, so their reactive groups are brought close together* and stay together long enough for the reaction to proceed. This eliminates randomness of collision in free solution (increases Z) (Figure 8.6).
*In the case of apparently unimolecular reactions, it’s a reaction between the single substrate and reactive amino acid side chains in the enzyme:
Orientation effect: even if two molecules manage to meet by random collision, their reactive groups are not necessarily pointed in the right direction to proceed with the reaction.
Since binding of substrates by enzymes involves very specific interactions, substrates of enzymes are precisely positioned and reactive groups are well aligned. This eliminates randomness of substrate orientation (increases p) (Figure 8.7).
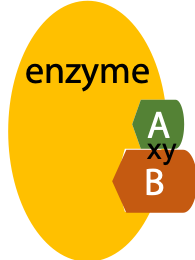
Randomness can be represented by entropy, so the proximity effect and orientation effect both reduce the activation entropy of the reaction.
Proximity effect is the elimination of translational entropy, while orientation effect is the elimination of rotational entropy. These entropies can be calculated at any given temperature. This allows us to estimate that a theoretically perfect enzyme could speed up a reaction by 105-fold by the proximity effect, and by 105-fold by the orientation effect. Overall, this is a 1010-fold speed-up for the combination of two effects. These two effects account for much of the observed speed up in enzyme catalysis.
How enzymes decrease Ea (Figure 8.8):
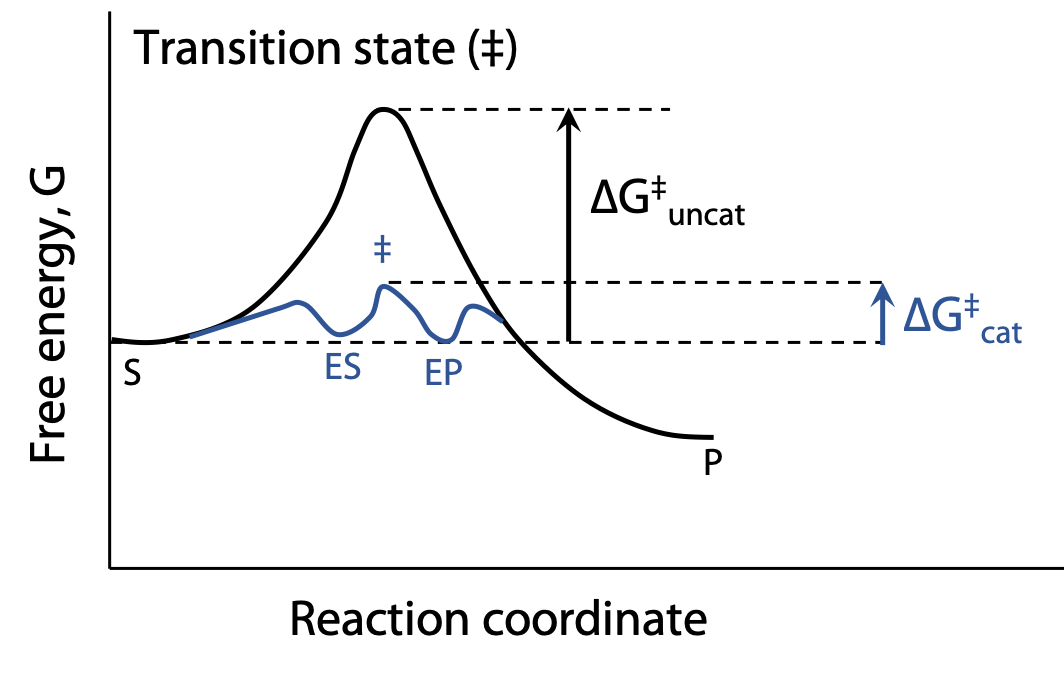
Chemical catalysis – the enzyme provides a reaction pathway that’s better than the uncatalyzed reaction. At 298 K, reducing Ea by 5.7 kJ increases rate 10-fold.
For example, examine the hydrolysis reaction:
X-CO-NH-Y + H2O X-CO2– + +NH3-Y
This overall equation for hydrolysis shows the addition of H2O to break an amide bond. With H2O alone, the reaction is incredibly slow, because the :O in H2O is a poor nucleophile and an excessively weak acid. Although O has two lone pairs, it’s very electronegative and reluctant to share its electrons. The reaction is radically speeded up by using OH¯, a much better nucleophile due to the excess negative charge, or H3O+, an excellent proton donor.
However, most biochemical reactions happen close to pH 7, so strong acid or strong base are ruled out.
(Nor can we raise the temperature, the chemist’s usual trick for speeding up reactions, reducing Ea/RT by increasing T).
Enzymes speed up reactions at normal pH and temperature.
Nucleophilic catalysis: reaction is initiated by a nucleophile on the enzyme, e.g. CysSH, or His or Asp- or Glu-, more rarely TyrOH, SerOH or LysNH2. Nucleophiles act by donating a lone pair to an electron deficient C atom in the substrate (e.g. C=O groups).
Electrophilic catalysis: an electrophile is a species, which reacts by attracting electrons from a reactant. There are no really excellent electrophiles among the amino acids, but what the enzyme might do is to recruit a non-amino acid helper molecule called a prosthetic group. The prosthetic group is bound into the enzyme’s active site like the substrate. The metal Zn2+ sometimes functions in this way. It is found in the active site of carboxypeptidase A, an enzyme that hydrolyzes a single amino acid from the C-terminus of a peptide chain.
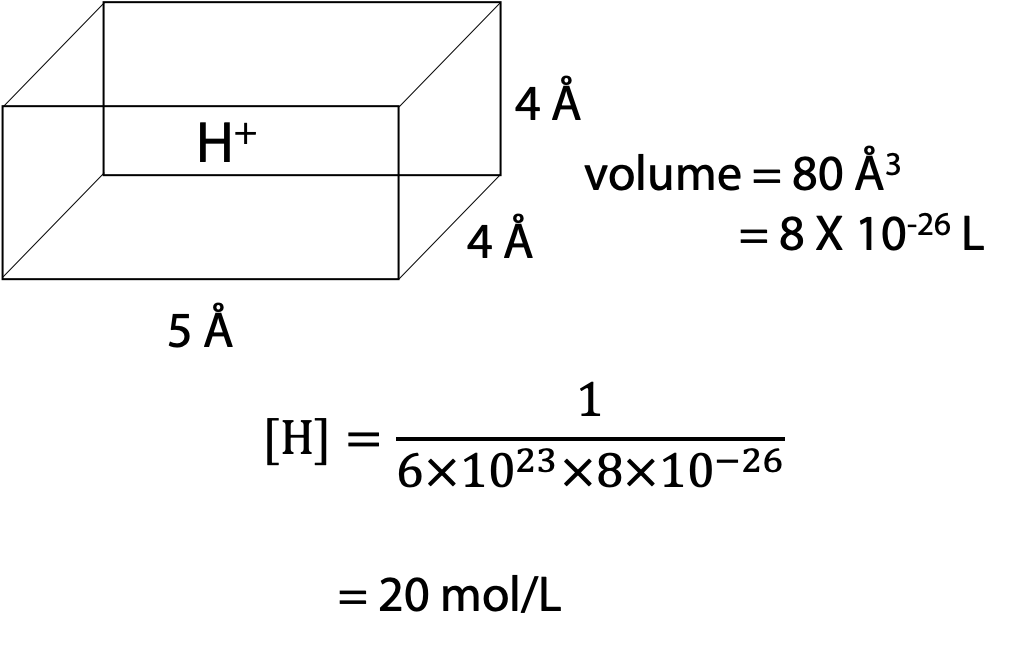
General Acid and General Base catalysis: catalysis by weak acid or weak base functional groups that donate a proton to or steal a proton from the substrate (as opposed to specific acid or base catalysis, which involves specifically H3O+ or OH¯).
The enzyme can position an acidic or basic group in a confined space in close proximity to the substrate. If a single H+ donor is localized in a small volume, it behaves as if the concentration of the reactive group is very high, without altering the pH of the general environment (Figure 8.9).
Each of the above effects can contribute a factor of about 102-fold to speed up the overall enzyme catalyzed reaction.
Transition state stabilization
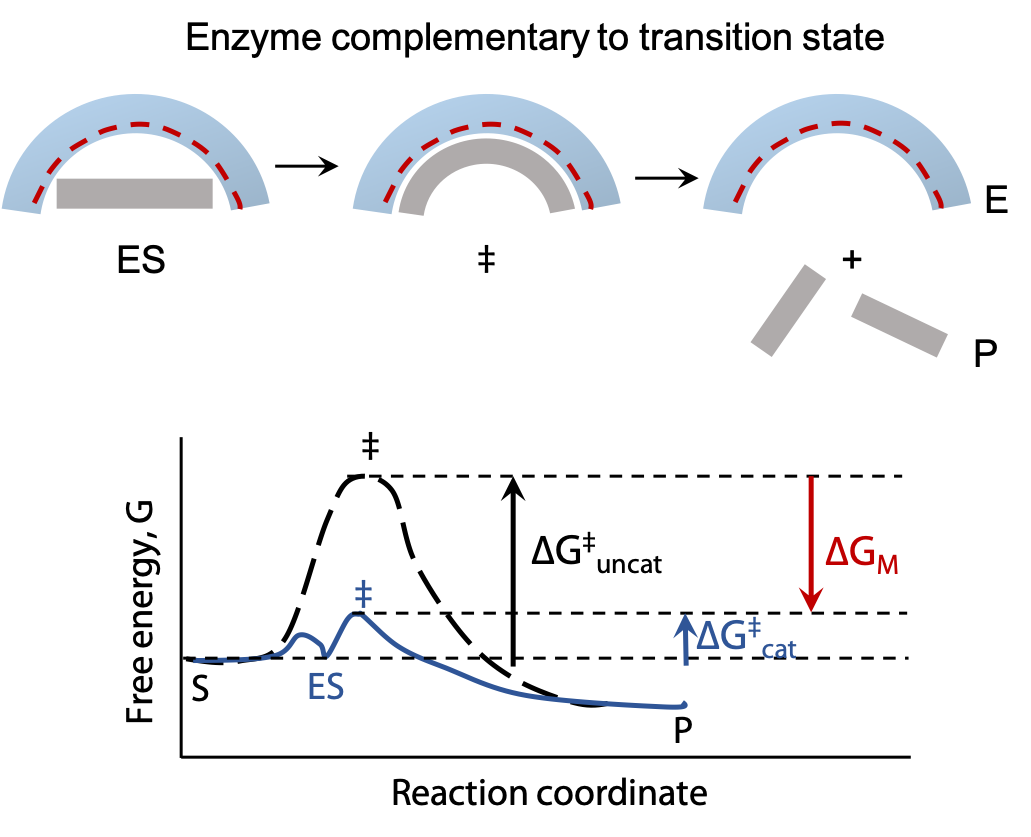
Transition state stabilization: all reactions pass through a transition state which represents an energy maximum; Ea is the energy needed to get up to the transition state. Enzymes can reduce Ea by providing a different chemical pathway through the reaction.
By shaping the active site of the enzyme so it fits the transition state even better than the substrate, Ea can be significantly lowered. When the substrate binds, the enzyme may stretch or distort a key bond and weaken it, so that less activation energy is needed to make the bond break at the start of the reaction. In many cases, the transition state of a reaction has a different geometry at the key atom (e.g. tetrahedral instead of trigonal planar). By optimizing binding of a tetrahedral atom, the substrate is helped on its way to reaction.

Self-assessment Questions
1.
2.