12 Lipids: fatty acids, triglycerides and phospholipids
Biological lipids are a structurally diverse group of molecules. Lipids, as a class of molecules, are not defined by their chemical structures, but by their common chemical property: hydrophobicity.
Lipids are those biomolecules that partition into the organic solvent phase when we perform a two-phase extraction of a cell extract. (Typically, the organic solvent used for the extraction is a 2:1 mixture of chloroform to methanol).
Biological functions of Lipids
Lipids are highly diverse with respect to both structure and function. Here is a simple listing of some of their major biological functions.
- Energy Storage – Triacylglycerols (Fats and oils)
- Structural elements of biological membranes – Phospholipids and sterols
- Enzyme cofactors – Coenzyme Q (mitochondrial electron transport chain)
- Signalling molecules – Paracrine hormones (prostaglandins, diacylglycerols), Steroid hormones
- Pigments – Colour of tomatoes, carrots, pumpkins, some birds
- Vitamins -Vitamins A and D (Hormone precursors), E (antioxidant) and K (blood clotting)
- Insulation from environment – Fats
- Waterproof coating – waxes
Lipids can occur covalently linked to other classes of biomolecules
Glycolipids, such as sphingolipids and gangliosides, contain both sugar and lipid portions and are important constituents of cell membranes. For example, the human blood groups (O, A, B) are defined by the glycolipids displayed on the outer surfaces of blood cells.
Lipoproteins – Many proteins also incorporate covalently bound lipids. HDL, LDL, and VLDL are plasma lipoproteins that are associated with cardiovascular health and disease.
Glycolipids and lipoproteins will not be discussed in this course.
We are only going to look at three fundamental classes of lipids in this course: fatty acids (which are the simplest lipids) and two classes of lipids that are derived from fatty acids.
1. FATTY ACIDS
– building blocks of many complex lipids
– central intermediates in metabolism
but free fatty acids present in trace quantities.
2. TRIACYLGLYCEROLS
– storage fat
3. PHOSPHOGLYCERIDES
– the major lipids in membranes
Fatty Acids
LABORATORY-RELATED CONTENT
The information discussed in this section is related to Lab 4.
Fatty acids are carboxylic acids with hydrocarbon chains ranging from 4-36 carbons. The most abundant fatty acids in cells have even numbers of carbon atoms.
Fatty acids with no double bonds between carbons in the chain are described as saturated fatty acids. Saturated means “containing as many H atoms as the molecule can hold”. That is, all the C atoms in a saturated fatty acid (other than at the ends) are -CH2 -. Fatty acids with one (monounsaturated) or more (polyunsaturated) double bonds (triple bonds are not common) in the chain are called unsaturated fatty acids.

The carbon atoms could be numbered 1, 2, 3 … starting at the carboxylic acid; or in Greek, alpha, beta, gamma … starting at the carbon that bears the carboxylic acid (the same system that is used to label the carbon atoms in amino acids); or, for some purposes, we identify the carbon atom positions relative to the terminal methyl carbon, which we call “omega” (ω). All of these numbering systems are in use (Figure 12.1).
Nomenclature
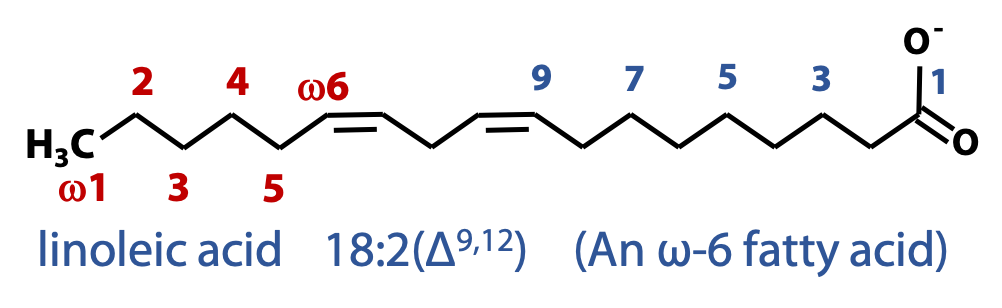
A simplified nomenclature used to describe fatty acids specifies the number of carbons in the chain followed by the number of double bonds, separated by a colon. e.g., 12:0 (12 carbon saturated fatty acid), 18:2 (18-carbon fatty acid with two double bonds) (Figure 12.2).
The positions of double bonds in unsaturated fatty acids are specified relative to the carboxyl carbon (C-1) by superscript numbers following Δ (Figure 12.3).
An alternative convention is to specify the position of double bonds relative to the methyl carbon (ω). This convention is used to name the fatty acids that carry double bonds between the third and fourth carbon atoms from the methyl carbon (ω3) and those that have double bonds between C-6 and C-7 from the methyl group (ω6). These fatty acids play an important role in human nutrition.
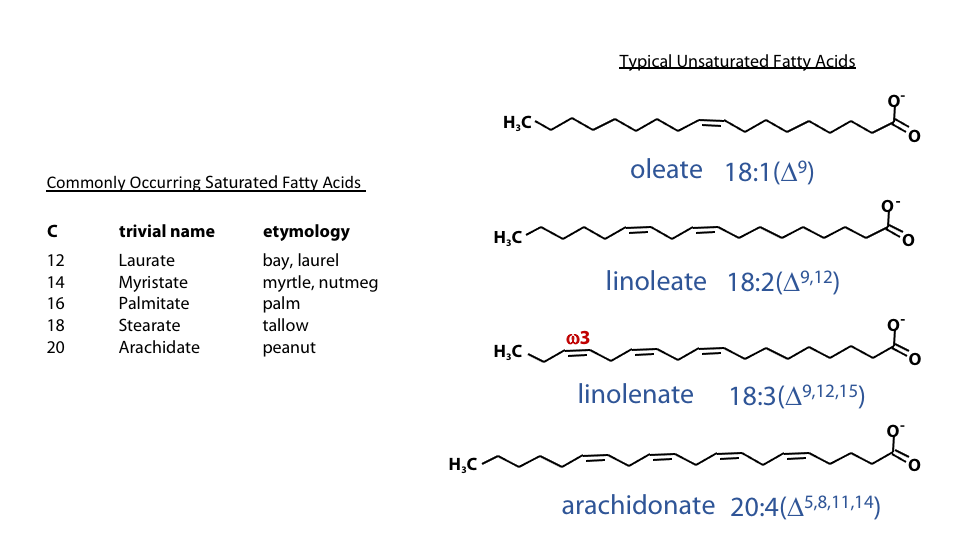
Features of commonly occurring fatty acids
-
Figure 12.4 PUFA usually have methylene-bridged double bonds (top) rather than conjugated double bonds (bottom) Have an even number of carbon atoms.
- Are unbranched
- Polyunsaturated fatty acids (PUFA) usually have the double bonds separated by a methylene carbon (methylene-bridged). Which has the bond pattern: double-single-single-double (Figure 12.3).
Conjugated double bond patterns (double-single-double-single) are certainly found in biomolecules (for example, see the structure of carotenoids in Stryer 19.5.2) but they are not common in fatty acids.
-
Figure 12.5 Cis-double bonds produces a kink in the chains of fatty acids. The unsaturated double bonds in naturally-occurring fatty acids are usually found in the “cis” configuration. This introduces a kink in the chain (Figure 12.4). “Trans” fatty acids are uncommon. However, the process of partial hydrogenation of oils, which is used to make them “harder” in manufacturing margarine and similar products, can also isomerize the double bonds, generating large amounts of “trans fats”. A trans double bond allows a given fatty acid to adopt an extended conformation. These synthetic fats are believed to have serious negative effects on cardiovascular health.
Solubility and melting point of saturated fatty acids
LABORATORY-RELATED CONTENT
The information discussed in this section is related to Lab 4.
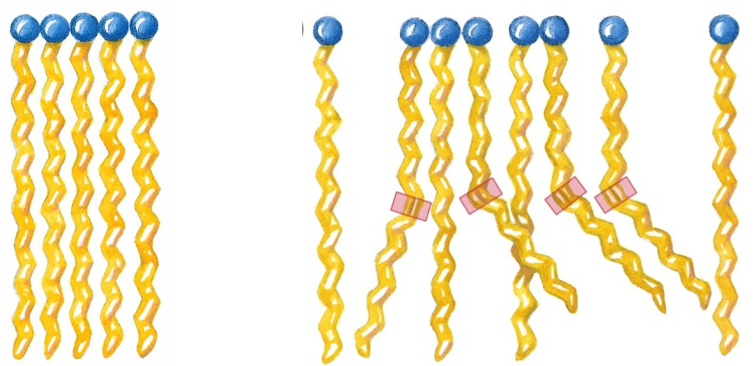
The physical properties of fatty acids are determined mainly by the length and degree of unsaturation of the hydrocarbon chain. i.e., Unsaturation drastically lowers the melting point of a fatty acid, and thereby increases the fluidity of a lipid bilayer. This fact underlies a great deal of lipid and membrane biochemistry!
Saturated fatty acid chains adopt extended conformations (Figure 12.6). In this conformation, they pack together tightly with atoms all along the length of the hydrocarbon chains forming Van der Waals contact with atoms of neighboring molecules. As the chain length increases, the melting point increases, while the solubility decreases.
Melting Point and Double Bonds
The kinks formed by the cis double bonds of unsaturated fatty acids (Figure 12.6, right) do not allow the molecules to pack as tightly together as the saturated fatty acids. This leads to much weaker interactions between molecules. Due to the fact that smaller thermal energy is required to disrupt these interactions, the melting temperature of these fatty acids is drastically lowered.
Stearic acid 18:0 = +70˚C
Linoleic acid 18:2(Δ9,12) = -5˚C
Due to their extended nature, trans fatty acids can pack more regularly, leading to show higher melting points than cis forms.
e.g. 18:0 = 70˚C; 18:1 (cis) = 13.4˚C; 18:1 (trans) = 45˚C
Derivatives of Fatty Acids:Esters and anhydrides
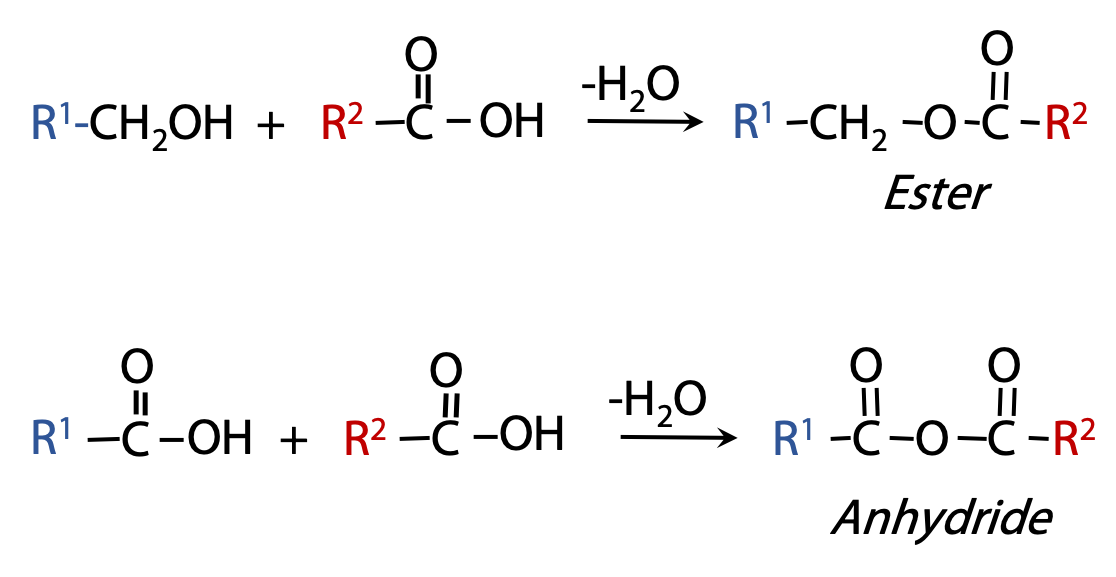
Carboxylic acids can combine with alcohols to form esters; and they can combine with acids to form acid anhydrides (Figure 12.7). These are both condensation reactions; conversely, the esters and anhydrides can be hydrolyzed to release the fatty acids.
Free fatty acids never persist for long in cells; they are usually converted into ester or anhydride derivatives.
Triacylglycerols (TAGs, triglycerides)

Triacylglycerols are formed by linking three fatty acids to a glycerol molecule through ester linkages (Figure 12.). The three fatty acids in a TAG could be the same (simple TAGs) or a combination of two or three different fatty acids (mixed fatty acids).
Triacylglycerols (“acyl” just means “an acid derivative”) are the most important storage form of fatty acids. TAGs are the major constituent of bulk fats and oils, including the human body’s depot fat. Notice how highly hydrophobic they are – the polar carboxylic acids of the fatty acids are tied up as (less polar) esters. That’s why fats and oils are insoluble in water!

Most natural fats are complex mixtures of simple and mixed triacylglycerols (Figure 12.9). As in the case of fatty acids themselves, the physical properties of the compounds that contain fatty acids are also determined by the length and the degree of saturation of the hydrocarbon chains of their constituent fatty acids. The higher the percentage of longer chain (C16 and C18) saturated fatty acids, the higher the melting temperature of the TAG. TAGs of animal origin are generally called “fats” and their melting temperatures are high due to the higher percentage of longer chain saturated fatty acids in them. Plant “oils” have lower melting temperatures as they contain a higher percentage of unsaturated fatty acids.
Phosphate derivatives of fatty acids
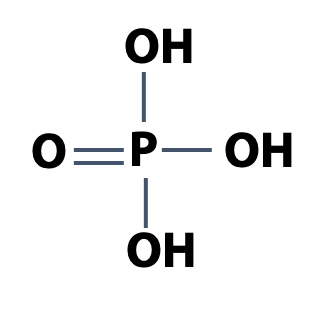
Phosphorus is one of the essential elements of life. Recall that phosphoric acid is H3PO4 (Figure 12.10). That’s a strong acid. Phosphoric acid is a triprotic acid. At neutral pH, the second proton is about half-dissociated. We write Pi to represent an equilibrium mixture of H2PO4 – and HPO4-2, the usual state of phosphate in the cell (Figure 12.11).
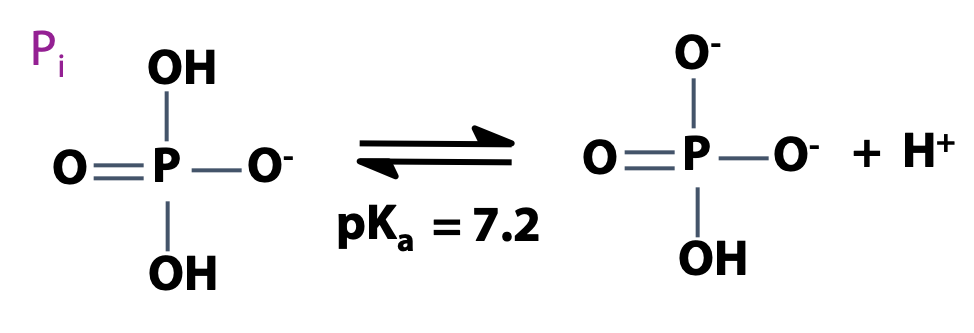
Phosphorylation adds negative charges to a molecule, increasing its water solubility. Phosphate derivatives are found throughout biochemistry — they are very important!
Some examples are:
Phospholipids
Sugar-phosphates
Many proteins
Nucleotides/ RNA/ DNA

Phosphoric acid can react with alcohols and acids to form phosphate esters and phosphoanhydrides (Figure 12.12). Phosphoric acid can react with organic compounds to form derivatives. This is analogous to the way that organic acids (e.g. acetic acid) react with organic compounds. Acetic acid + ethyl alcohol gives an ester (ethyl acetate). Acetic acid + acetic acid gives an anhydride (acetic anhydride). These are condensation reactions (removal of the elements of water). In the same way, phosphoric acid + an alcohol gives a phosphate ester; and phosphoric acid + an acid gives a phosphoanhydride.
Glycerophospholipids
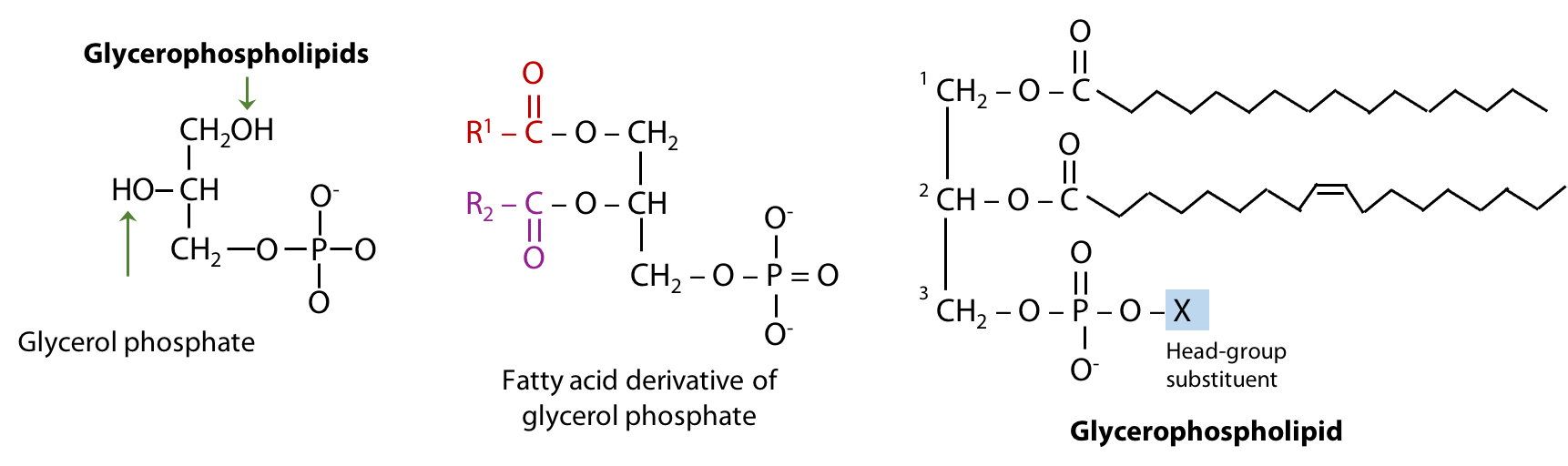
Glycerophospholipids are the major constituents of biological membranes. They are derivatives of glycerol phosphate formed by linking carbon atoms 1 and 2 of glycerol phosphate to two fatty acids through ester linkages and carbon atom 3 to a highly polar or charged alcohol group (X) through a phosphodiester linkage (Figure 12.13). The two fatty acids esterified to glycerol phosphate form the “tail” portion of a glycero-phospholipid, while the highly polar or charged group (X) attached through the phosphodiester linkage forms the “head” portion of the molecule.
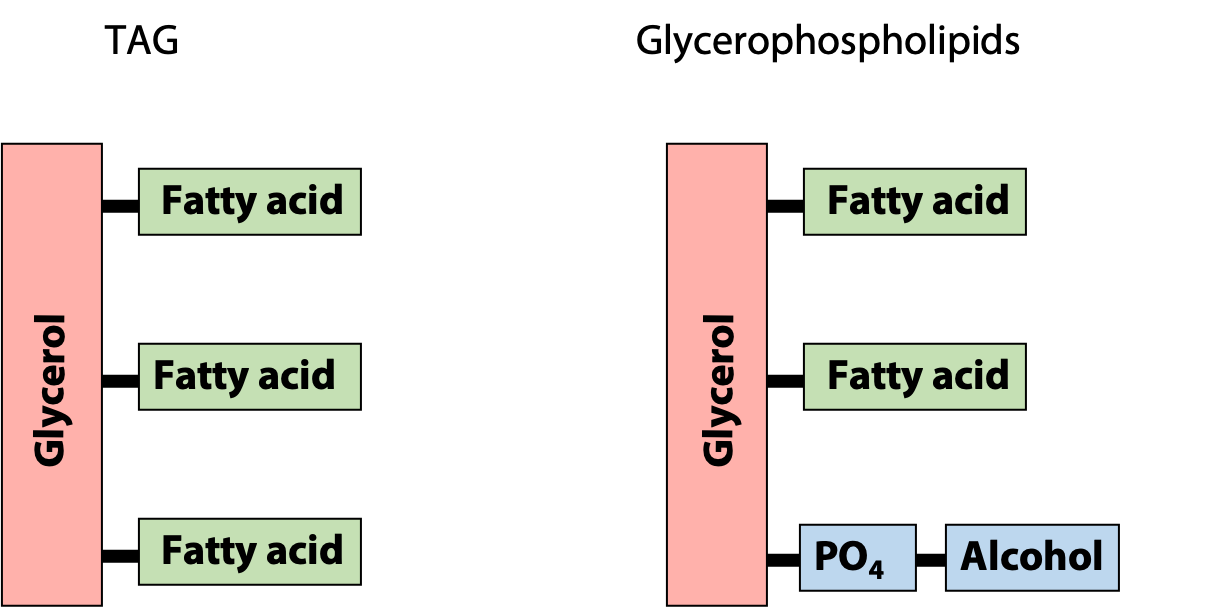
The polar “head group” of the glycerophospholipid makes it amphipathic, i.e., it combines both hydrophilic and hydrophobic properties. This makes the physical properties of glycerophospholipids very different from those of the insoluble storage fats – it is the reason why glycerophospholipids form lipid bilayers. Notice the similarities and differences between TAGs and glycerophospholipids (Figure 12.14).
Major classes of Glycerophospholipids


Table 12.1 shows the four major classes of glycero-phospholipids. The alcohol group linked through the phosphodiester linkage in phosphatidylcholine is choline (N,N,N-trimethylethanolamine). Phosphatidylcholine is commonly called lecithin (Figure 12.15). Phosphatidylcholine, like all other glycerophospholipids, represents a class of lipids rather than a single molecule. Different combinations of fatty acids at carbon atoms 1 and 2 of the glycerol phosphate moiety correspond to different phosphatidylcholine molecules. We already saw this situation when we looked at TAGs.
The second class of glycerophospholipid common in most membranes isphosphatidylethanolamine, in which the head group is formed by the alcohol, ethanolamine (OH-CH2-CH2-NH3+). The alcohols esterified to the phosphate group in phosphatidylserine and phosphatidylglycerol are serine and glycerol respectively. The composition of different plasma and organelle membranes are shown in Figure 12.16. As you can see, phosphatidylcholine (PC) and phosphatidylethanolamine (PE) are the major constituents of most membranes.
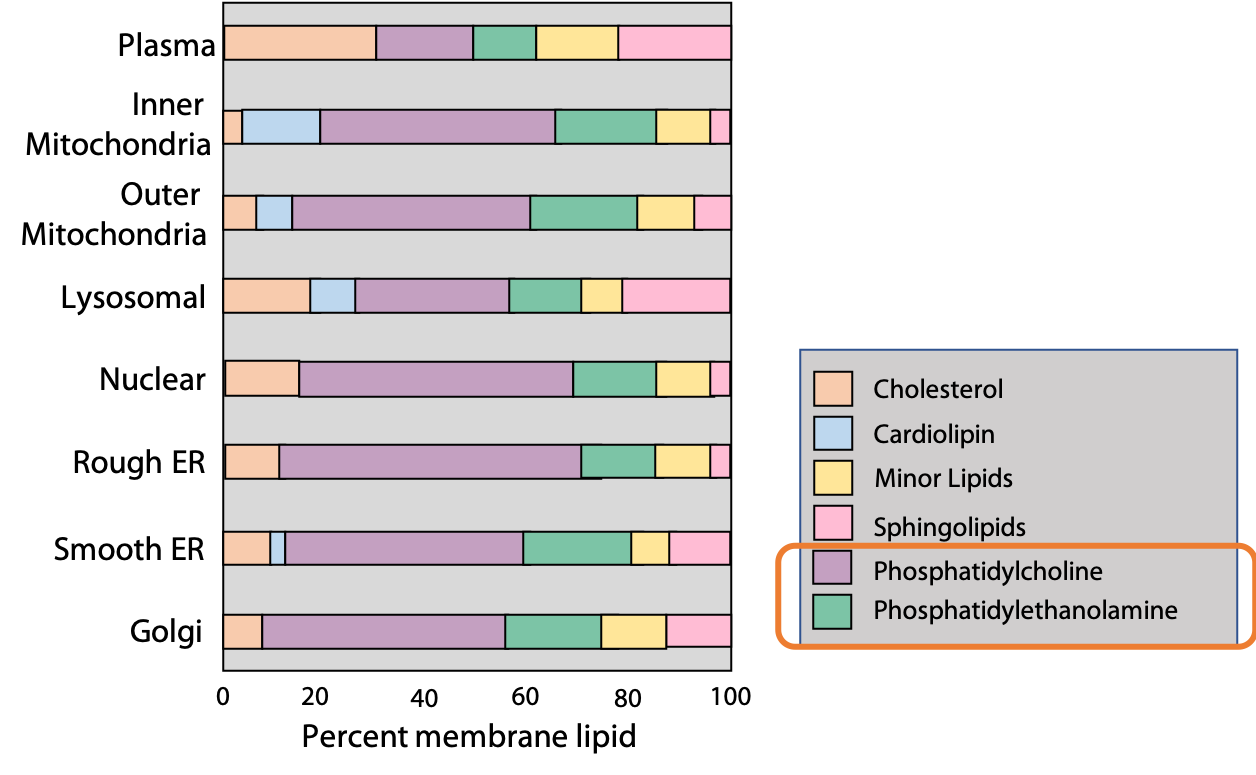
Lipids aggregate spontaneously to form complexes when dispersed in water
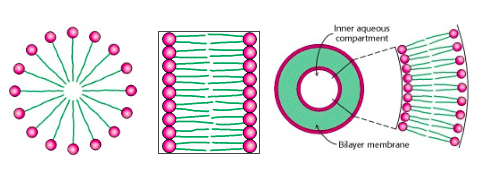
Because lipids are hydrophobic, they do not dissolve in water to form aqueous solutions – at least, not at appreciable concentrations. Instead, they aggregate spontaneously to form complexes, which may range in size from a few lipid molecules to thousands or more (Figure 12.17). The nature of the aggregate depends on the chemistry of the lipid and on the environmental conditions, such as temperature and the presence of other solutes. Fatty acids (and some other lipids) aggregate to form roughly spherical micelles. Micelles are the smallest and simplest lipid aggregates, with diameters of roughly 3 up to a few hundred nm. The cloudy appearance of soapy water is due to the scattering of light by micelles. (Some proteins also form micelles; micelles of casein protein are what make milk look “milky”.)
Phospholipids spontaneously form aggregates that are much larger than the nm-scale micelles. The hydrophobic tails of phospholipids are too bulky to pack tightly together in micelles (Fig above). Instead, phospholipids typically aggregate into bilayers (a double layer of phospholipids), which spontaneously fold back on itself to form vesicles or liposomes, with diameters as large as 1 micron or more. A liposome is a roughly spherical structure and can be considered as a sort of “proto-cell” – a bilayer of lipid membrane surrounding an aqueous compartment.
Synthetic liposomes are now being used for delivery of drugs. A liposome (e.g., injected intravenously) can fuse with the lipid bilayer of a target cell membrane and thereby deliver its contents into the cell. This provides a means for delivery of polar, water-soluble drugs that would not normally be able to enter a cell.
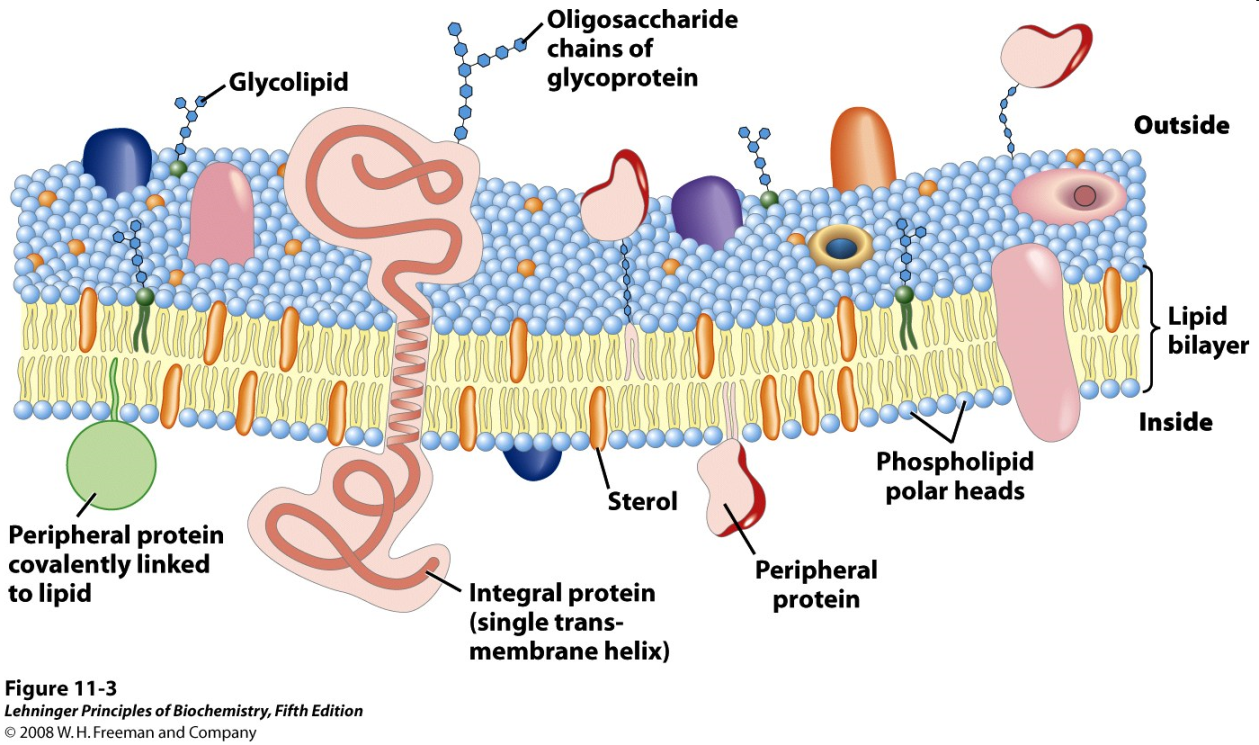
The lipid bilayer is the fundamental structure of the cell membrane. A real biological membrane; however, is a far more complex structure, with a high content of hydrophobic proteins, oligosaccharides, and other structural and functional features in addition to the fundamental phospholipid bilayer (Figure 12.18).
Analysis of Lipids
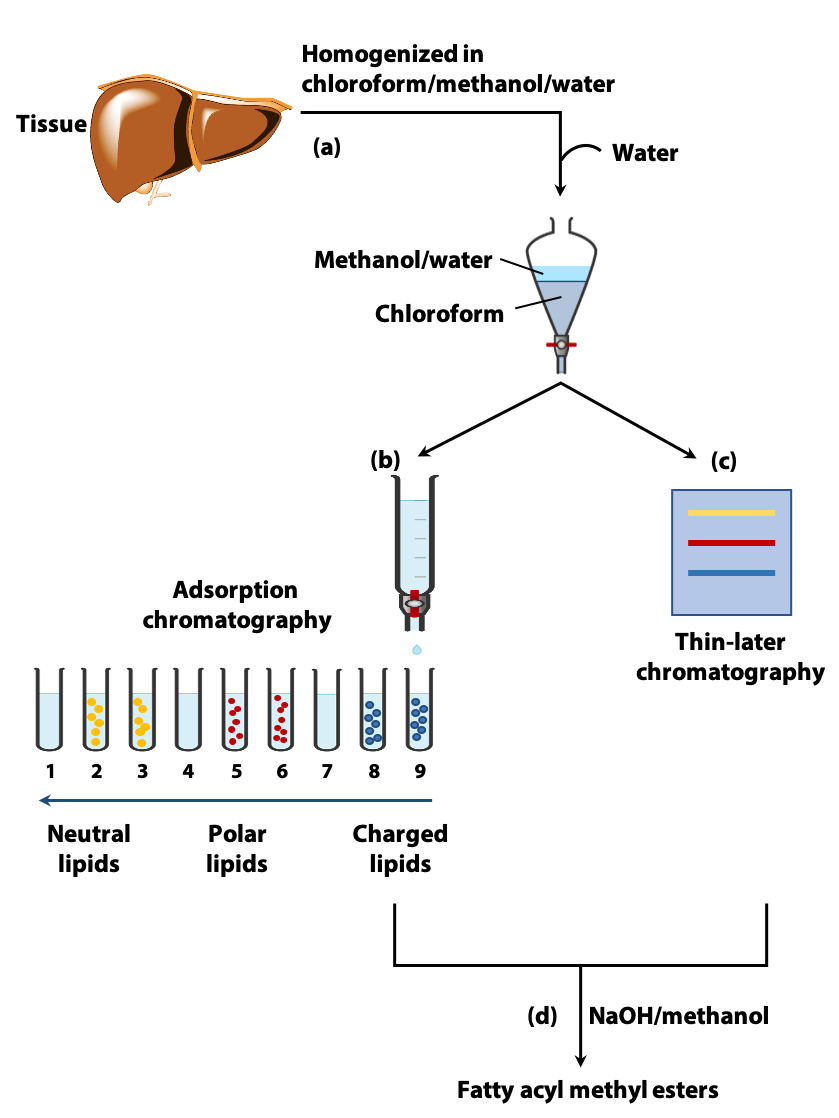
Due to their hydrophobicity, the extraction and fractionation of lipids require the use of organic solvents (Figure 12.19a). The type of organic solvent used depends on the type of lipid being extracted (e.g., triglycerides, phosphoglycerides). A commonly used extractant is a mixture of chloroform, methanol and water. The lipids in the homogenized tissue are separated into the chloroform layer, while the more polar molecules (proteins, sugars) partition into the methanol/water layer (a).
Once extracted, the different types of lipids in the chloroform phase can be separated by adsorption chromatography. This could be carried out on a column of silica gel through which solvents of increasing polarity are passed (b) or with the use of thin layer chromatography (less polar lipids moving farther than the more polar lipids on a silica gel coated plate) (c).
Adsorption chromatography separates the different types of lipids (e.g. TAG, phospholipids etc.). But, as we have discussed, the properties of these lipids depend on their constituent fatty acids. Therefore, it is important to determine their fatty acid composition. The fatty acid molecules are very similar in chemical properties and they cannot easily be separated by “classical” chemical methods.
Analysis of fatty acid composition:
-
Figure 12.19b One method of lipid analysis involves the use of gas-liquid (e) or high performance liquid chromatography. Transesterification – The fatty acids that are linked through ester linkages to TAG and phospholipids break away from them to form new ester linkages with methanol when mixed in a warm solution of NaOH and methanol. These methyl esters of fatty acids are more volatile (d).
- The fatty acyl methyl esters are separated on the basis of chain length and degree of saturation by gas-liquid chromatography (GLC) (Martin and Synge, Nobel Prize in Chemistry, 1952) or high performance liquid chromatography (HPLC) (Figure 12.19b, e & f).
- Combination of GLC and HPLC with mass spectrometry allows definitive identification of the separated fatty acids.