13 Carbohydrate Chemistry: simple sugars, rings and glycosides
Carbohydrates, in common speaking, are called “sugars”. Sugars are the most abundant biomolecule on earth. Unlike the proteins, which are built from a limited set of building blocks (the amino acids), the number of possible sugar molecules is very large. However, only a few of these sugars are commonly found in cells, and for the purposes of this course, we only need to consider a few specific sugars. We will be looking at the general principles of carbohydrate chemistry.
Carbohydrates are of great importance in energy metabolism, and are essential components of nucleic acids (RNA and DNA). They are also found attached to many proteins (“glycoproteins”).
Sugars are also called saccharides. Simple sugars, with only one sugar unit, are called monosaccharides. Larger sugars, built from two, three …. many monosaccharide units are called disaccharides, trisaccharides … polysaccharides. All monosaccharides share some chemical properties: they are very water-soluble, poorly soluble or insoluble in organic solvents such as ether or hexane, colorless, and, at least in most cases, sweet to the taste and have the approximate formula (CH2O) n.
What are sugars?
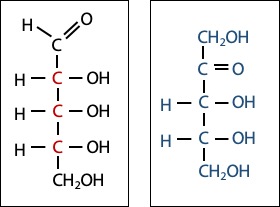
Monosaccharides combine two organic chemical functional groups: they have a carbonyl (C=O) group, which is either an aldehyde (with the carbonyl carbon bonded to one carbon atom and one hydrogen atom) or a ketone (with the carbonyl carbon bonded to two other carbon atoms), and they also have at least two carbons bearing hydroxyl (alcohol) groups (-C-OH). Therefore, sugars are polyhydroxy aldehydes or polyhydroxy ketones. The aldehyde-sugars are called aldoses and the ketone-sugars are called ketoses (Figure 13.1). Most sugars have names that end in “ose”, such as glucose and sucrose.
The chemical properties of sugars; therefore, reflect the properties of alcohols and ketones/ aldehydes – but, as we will see, they also exhibit some special properties that reflect the presence of both carbonyl and alcohol functional groups within a single molecule.
The simplest monosaccharides contain three carbon atoms (Trioses)
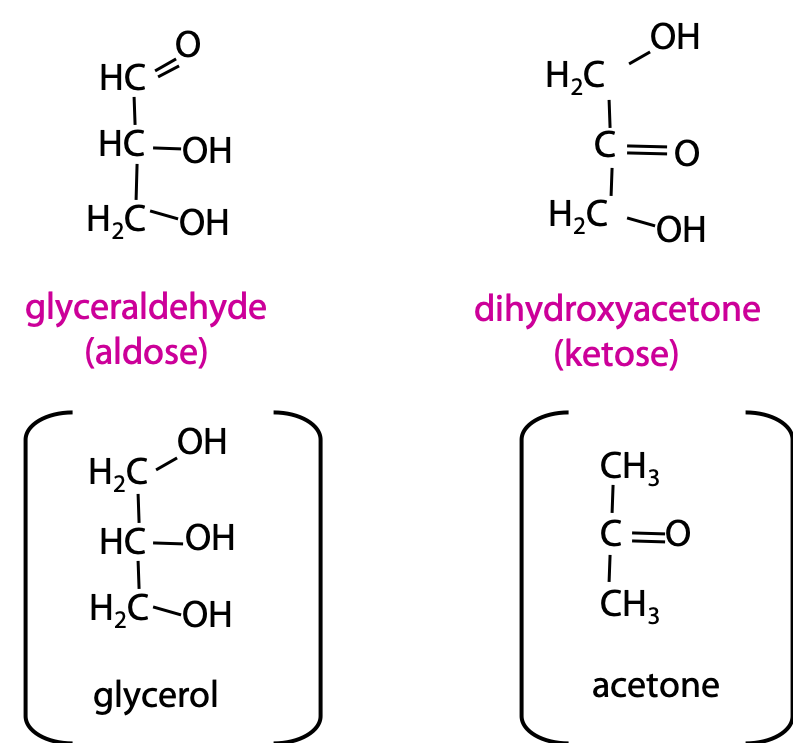
The simplest sugars contain three carbon atoms: one carbonyl group and two carbons bearing hydroxy groups. We call these sugars “trioses” (three-carbon sugars). There are two trioses: the aldose glyceraldehyde and the ketose dihydroxyacetone. Figure 13.2 shows you how the name glyceraldehyde reminds you of the three-carbon alcohol glycerol (a molecule that we have already encountered, when we discussed fats), and the name dihydroxyacetone reminds you of the three-carbon ketone acetone, which is a common organic solvent that you use in the lab.
Emil Fischer (1852-1911): Nobel Prize in Chemistry 1902
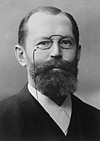
Much of our understanding of carbohydrate chemistry goes back to the work of the German chemist Emil Fischer (Figure 13.3) and his students, in the late 19th century. Fischer studied the analysis, synthesis, and stereochemistry of simple sugars. He introduced much of the terminology and notation that we still use today.
Fischer Projection Formula
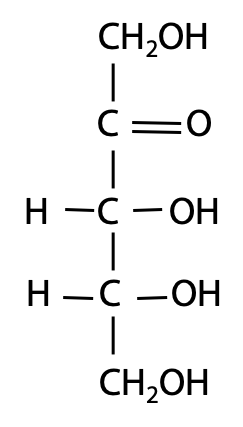
How do we draw sugar structures? Most of the carbon atoms are tetrahedral in geometry: not planar. So, if we want to draw sugars on a flat sheet of paper, we have to make some compromises. Following the lead of Emil Fischer, we can use a representation – the “Fischer projection formula” (Figure 13.4): Put the C atoms in a vertical row; the C atoms are numbered from top to bottom. Bonds in the vertical orientation are assumed to be bending back behind the page, and bonds in the horizontal orientation are assumed to be bending forward in front of the page.
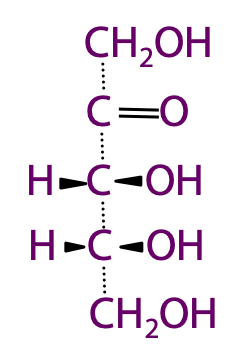
Perspective formula is another way of representing sugar structures. Solid wedge shaped bonds project in front and the dashed bonds point away (Figure 13.5).
Monosaccharides contain asymmetric carbon atoms: ENANTIOMERS
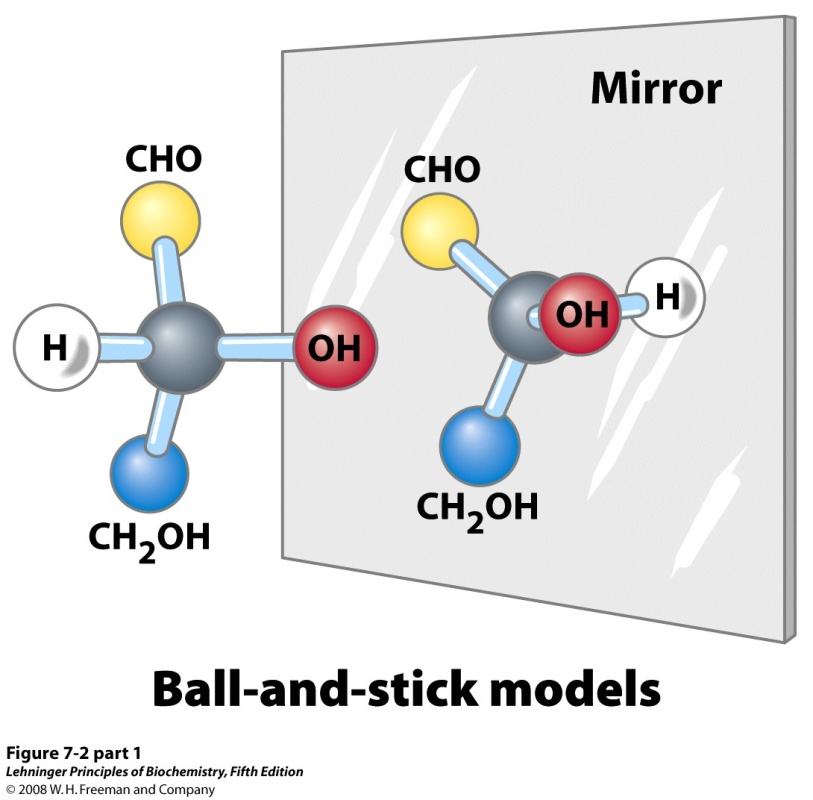
Sugar chemistry is dominated by the issue of chirality, i.e., asymmetry or “handedness” (Figure 13.6). A carbon atom with four substituents is tetrahedral, not planar. If the four substituents on a carbon atom in a molecule are all different, then the atom and the molecule are “chiral” (asymmetric). All monosaccharides except dihydroxyacetone contain one or more chiral carbon atoms. This gives rise to the occurrence of optically active isomeric forms (“left-handed” or “right-handed” form of a molecule). (Refer to first-year chemistry.) The “left-handed” and “right-handed” forms of a molecule are called enantiomers (mirror images).

Glyceraldehyde is the simplest aldose sugar. The central carbon atom in glyceraldehyde has four different substituents, so it is chiral (Figure 13.7). The “top” and “bottom” carbon atoms are not chiral. So, glyceraldehyde has two enantiomeric forms, which we call “D” and “L” glyceraldehyde.

(Historical note: The “D” and “L” designations go back to the issue of the rotation of the plane of polarization of a light beam: “D” stood for dextro (right) and “L” stood for “laevo” (left). The term “dextro” survives in the common name for the sugar D-glucose, which is often called “dextrose”). Look at the structure of dihydroxyacetone; you can see that it is not chiral (Figure 13.8).
Enantiomers have identical chemical properties such as melting points and water-solubilities. However, they differ in their optical activity; when we pass polarized light through solutions of the molecules, the plane of polarization is bent in opposite directions for the two enantiomers. It explains why we sometimes also refer to the “handedness” property of molecules as “optical activity”. (Historical note: The discovery, due to Louis Pasteur, that enantiomers have opposite “optical activities” was one of the first proofs that the tetrahedral carbon atom theory was valid.)
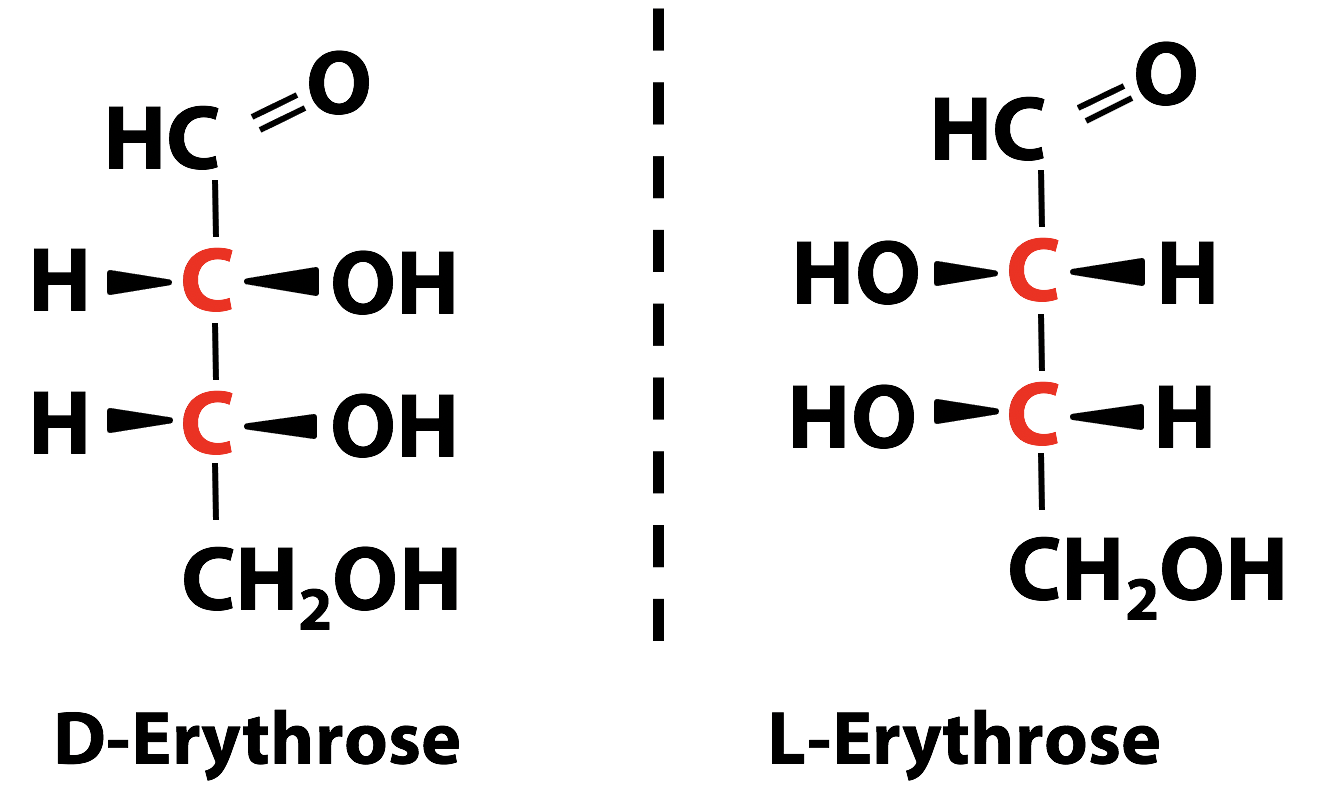
Sometimes a single molecule has more than one chiral carbon atom (the carbon atoms shown in red in the two sugar molecules Figure 13.9). The handedness in these cases applies to all the chiral carbon atoms in the molecule. These molecules can form stereoisomers whether they are mirror images of each other or not. If two molecules differ in handedness at each of the chiral carbon atoms, they are mirror images of each other and; therefore, are enantiomers.
Diastereomers

Monosaccharides with more than one chiral carbon atoms have stereoisomers that differ in handedness at some but not all chiral carbon atoms, which means they are not mirror images (enantiomers) but are diastereomers of each other (Figure 13.10). Diastereomers do not have identical chemical properties, because the spatial relationships among the atoms making up the molecule are different.
D and L designation of sugars with more than three carbon atoms
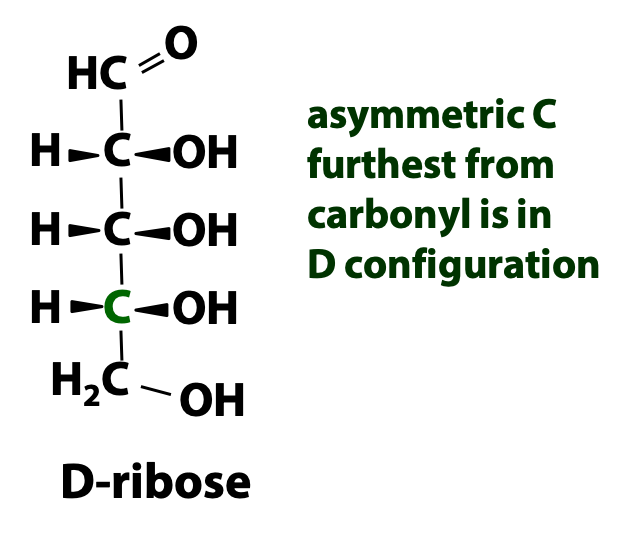
Most sugars in biology have more than three carbon atoms and have more than one chiral carbon atom. By convention, we will call a sugar a “D” sugar if the chiral (asymmetric) carbon atom furthest away from the carbonyl group has the same configuration as D-glyceraldehyde. This is illustrated by the molecule D-ribose, shown in Figure 13.11. Sugars with a configuration similar to L-glyceraldehyde are called “L” sugars. Most (but not all) naturally occurring sugars are D-sugars. For the remainder of the course, we are going to limit our discussion to the D-sugars only.
Epimers

We have already talked about diastereomers. When we have a pair of sugars that are identical except for the chirality (configuration) at one carbon atom, we call them “epimers”. Epimers are just a special case of diasteromers. Figure 13.12 shows an example: ribulose and xylulose are epimers at carbon #3. Why introduce this word? Well, quite often, chemical or biochemical processes will convert a particular sugar into an epimer; so it’s convenient to have this term to describe the relationship between the sugars.
A sugar with n chiral centres has 2n stereoisomers
The total number of stereoisomers formed by a molecule depends on the number of chiral carbon atoms the molecule contains. A molecule with one chiral carbon atom will have two stereoisomers; when two or more (n) chiral carbon atoms are present, there can be 2n stereoisomers. e.g., an aldose with 5 carbons has 3 chiral centres, will have 23 = 8 stereoisomers in total. Half these stereoisomers are D sugars and the other half are L sugars.
D-ketoses
Now we see why there are so many different simple sugars! One can make the carbon chains longer and longer — 3, 4, 5, 6, 7 … carbon atoms. Indeed, sugars with 7 C atoms are commonly found in biochemistry. Every time we add another C atom, the number of isomers doubles! Not all of these sugars are found in cells, but many of them are. And, for every C number (say, 6 carbons), there are both aldose and ketose sugars. (When we look at sugar cyclization, we will see that even a single sugar, like glucose, can exist in several different structural forms.)
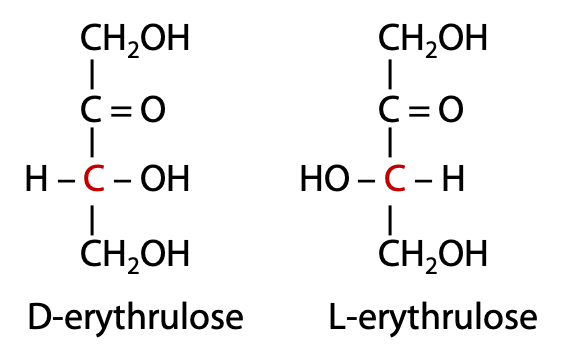
As we said earlier, dihydroxyacetone, a triose, is not chiral. Every carbon atom in a simple sugar bears a single -OH group, except for the carbonyl carbon. The terminal carbon atoms in a ketose are of the CH2OH type, while the non-terminal carbon atoms are H-C-OH. With four carbons, we have the tetrose called erythrulose, and it comes in D and L enantiomeric forms (Figure 13.13).

Figure 13.14 shows you the 5- and 6-carbon ketoses. Make sure that you understand their relationships. Do you understand, for example, why there are two pentoses (5-C sugars) and four hexoses (6-C sugars) in the chart?
With five carbons, we have two D-ketoses and two L-ketoses (not shown). The two D sugars are called ribulose and xylulose. Notice how the third carbon atom from the top can go in with the -OH group either “to the right” (ribulose) or “to the left” (xylulose). As a result, ribulose and xylulose are diastereomers: each has two chiral carbons; the configuration is the same at one of them (C-4) but opposite at the other (C-3). Specifically, they are epimers at carbon #3 (red box). Psicose and fructose are also epimers at carbon #3 (blue box). Psicose and sorbose are epimers at carbon #4 (green box). Note that all the sugars have the same configuration at the last chiral carbon (purple box): the -OH group is on the right. That’s because these are all “D” sugars.
D-Aldoses
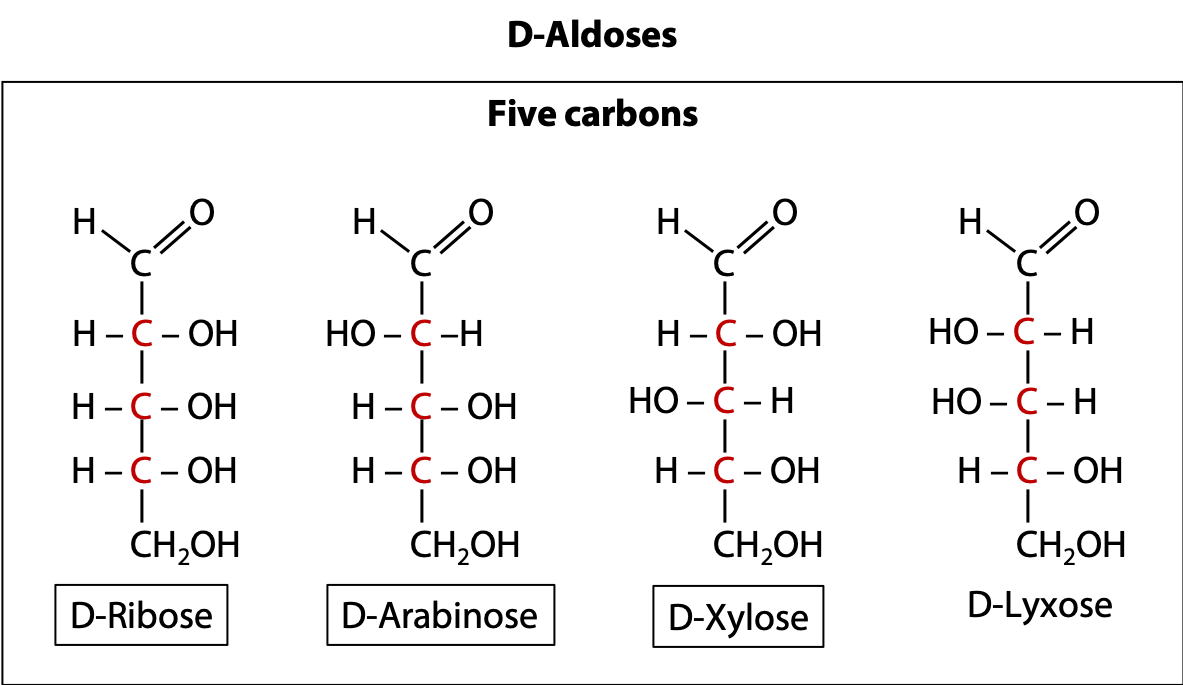
We just looked at two 5-carbon ketoses. In Figure 13.15 we see four 5-carbon aldoses. There are always twice as many aldoses, compared to ketoses, for a given number of carbons. Why? It goes back to the fact (see earlier) that there are two chiral forms of glyceraldehyde but only one form of dihydroxyacetone, which is a more symmetric molecule. Ribose, the first molecule shown above, is the sugar found in ribonucleic acid (RNA).
You are not expected to memorize the names or the structures of the sugars. And, although the number of sugars is large, they are built up in a logical and predictable way.
Hemiacetals and hemiketals
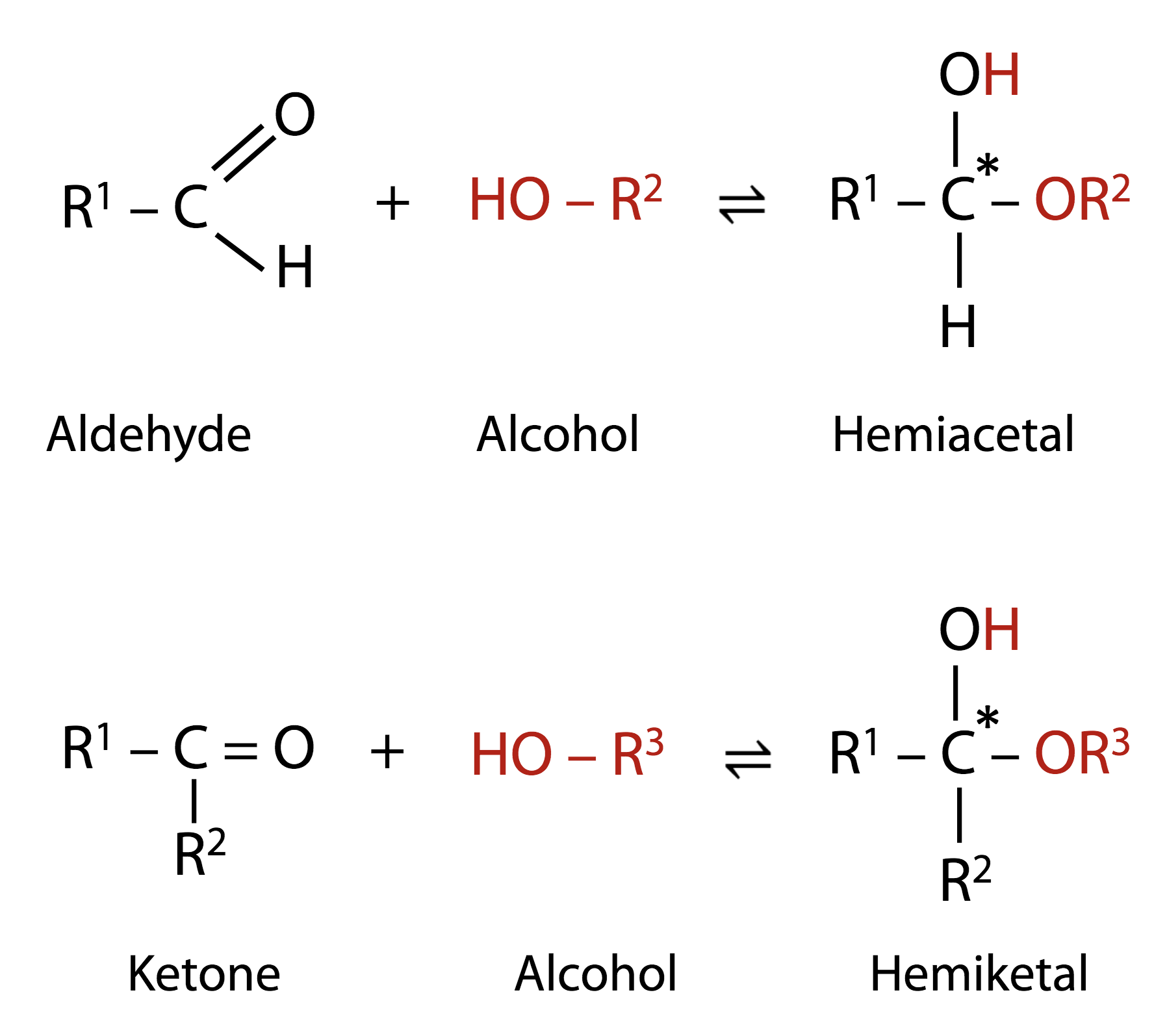
Aldehydes and ketones can react with alcohols, to produce “hemiacetals” and “hemiketals” (Figure 13.16). Any aldehyde (whether a sugar or not), can react with an alcohol, as shown in the figure, to form a “hemiacetal”. The same sort of thing happens with a ketone and an alcohol, giving a “hemiketal” product. Note also that, in the product, we have a new chiral carbon, as indicated by the asterisk. Therefore, in each case, we really get two products, with different configurations. Note that these reactions are reversible.
What does all this mean for sugars?
Cyclizations of sugars
Sugars have both alcohol and aldehyde or ketone functional groups. So they can react in the way we just looked at. But for sugars, the hemiacetal and hemiketal formation is intramolecular: the alcohol and the aldehyde (or ketone) are present in the same molecule. So the reaction goes much more easily. Hemiacetal and hemiketal formation turns sugars into ring structures.
Cyclization of glucose: ANOMERs
There are five different -OH groups in glucose. In terms of chemical reactivity, any one of them could react with the aldehyde. Geometrically; however, the -OH group at C-5 is the one in the best position to react. The OH group at C-5 reacts with the carbonyl carbon of the aldehyde group to form a stable six- membered ring (Figure 13. 17). This is by far the major reaction for glucose. Some other sugars form rings of different sizes.
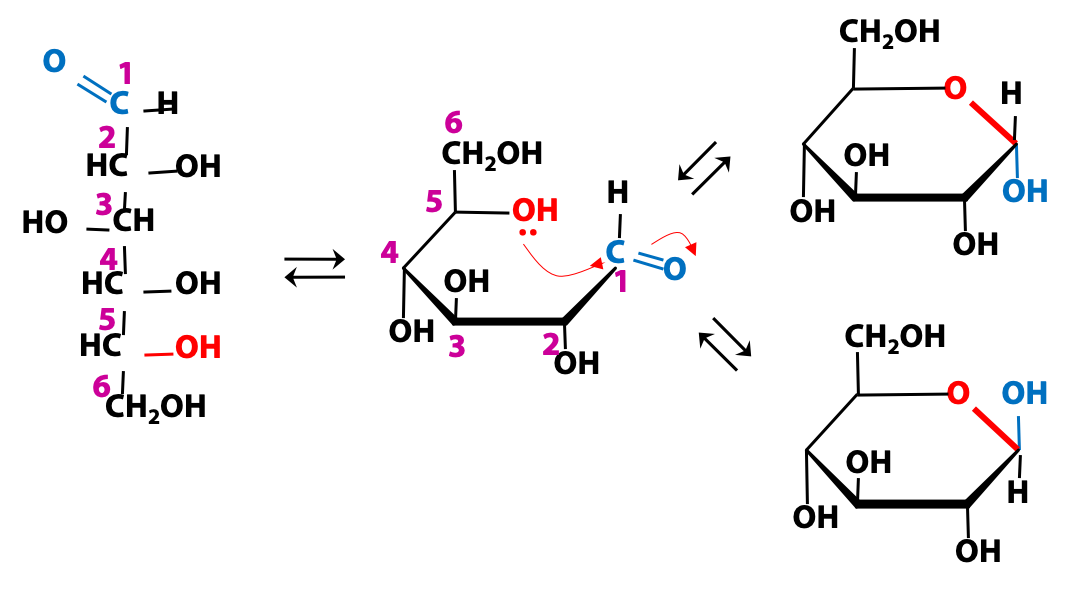
This reaction renders C-1, (the original aldehyde carbon atom) asymmetric (a chiral centre), leading to two possible epimers that can be formed. Epimers resulting from cyclization of a sugar are a special case and they are known as anomers. We distinguish them as “alpha” or “beta”. The carbon atom bearing the anomeric -OH group (C-1, for an aldose sugar), is called the “anomeric carbon”. (The anomeric carbon is the only carbon atom in the molecule that is linked to two different oxygen atoms).
Cyclization of a ketose: fructose
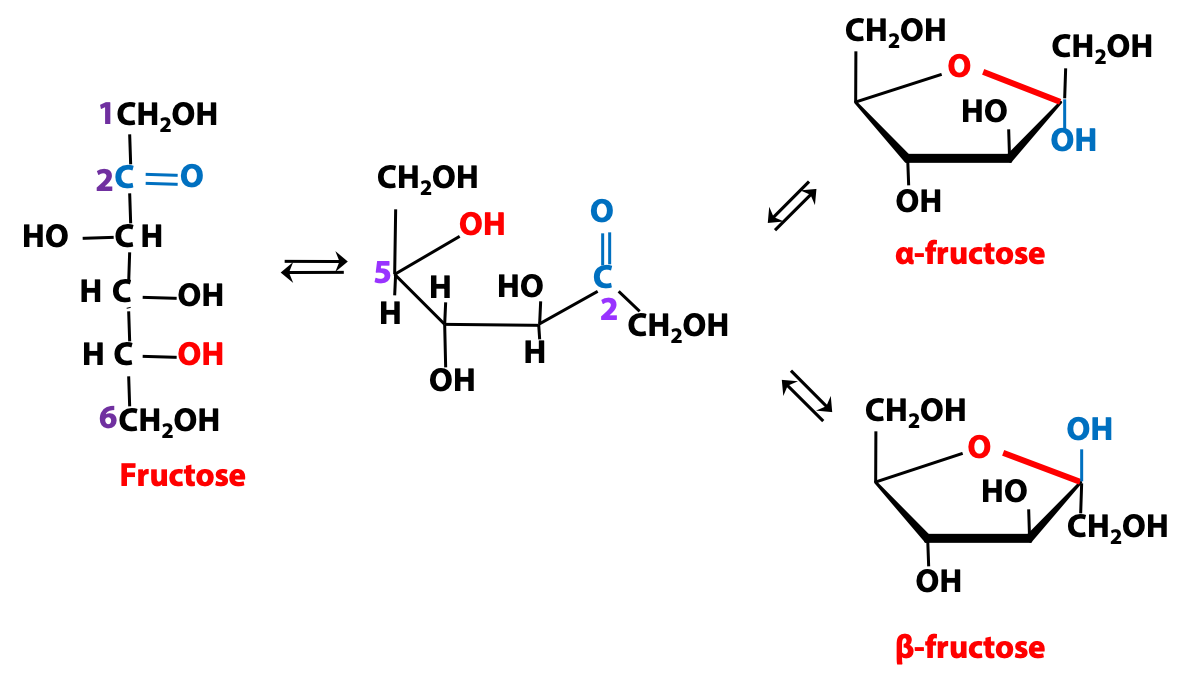
The cyclization chemistry for a ketose follows the same theme as for an aldose: the electrophilic carbonyl carbon atom reacts with the nucleophilic O of an -OH group (Figure 13.18). But now we have a carbon atom, rather than a hydrogen atom, attached to the anomeric carbon position.
Mutarotation
So, which form is “really” glucose – the linear (Fischer structure) molecule, or the ring structure that forms when the aldehyde makes a hemiacetal? Is the alpha or the beta form “really” glucose? Well, all three molecules are forms of glucose. When we prepare solid glucose, we usually get either the alpha or the beta form of the hemiacetal. But once we dissolve glucose in water, it slowly converts into an equilibrium mixture of the alpha, beta, and linear forms. This process is called “mutarotation”. When you dissolved glucose, the degree of rotation of the plane of polarized light, passed through the solution, would gradually change – So the “rotation” changed (“mutated”) – hence, “mutarotation”! With time, a solution of α-D-glucose and a solution of β-D glucose form identical equilibrium mixtures with identical optical properties (approx. composition ⅓ α-D-glucose, ⅔ β-D glucose and trace amounts of the linear form)
It’s important to understand that both the ring and the linear forms of glucose exist, because they have different chemical properties. For example, the linear form is an aldehyde but the ring form is not. This will matter, when we discuss glucose metabolism, later on.
When six-carbon sugars cyclize they form one of two ring forms; pyranose (6-membered) or furanose (5-membered)
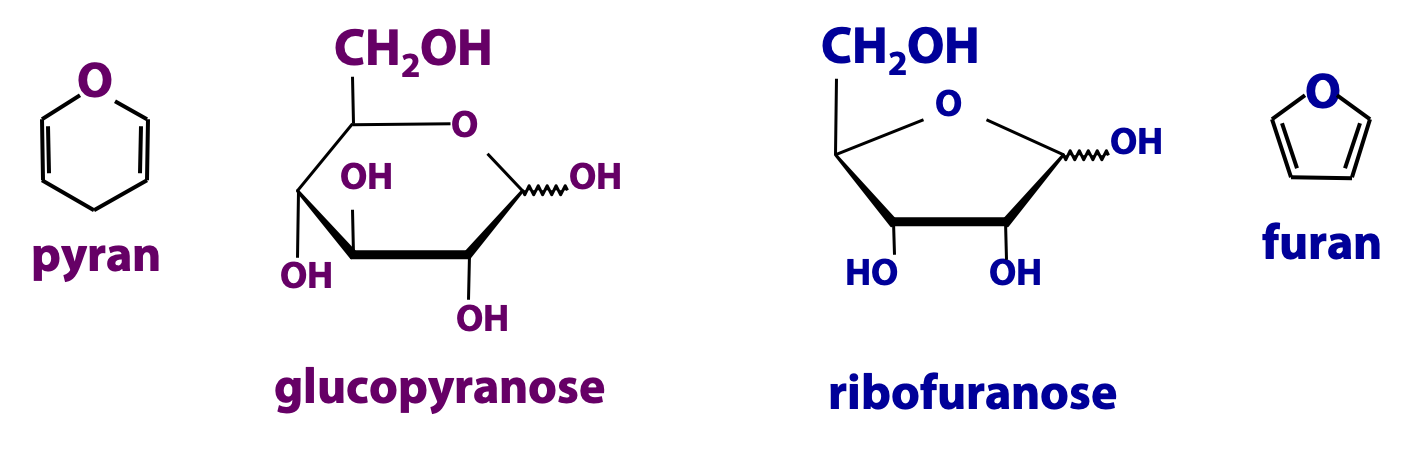
Do sugars always form 6-membered rings when they cyclize? No. Sometimes they form 5-membered rings. The 6-membered rings are called “pyranose” rings; the name is derived from the heterocyclic compound called pyran (Figure 13.19). The 5-membered rings are called “furanose” rings; the name is derived from the heterocyclic compound called furan. The size of the ring formed by a particular sugar depends on the relative thermodynamic stabilities of the various possible ring structures, and this depends on the particular geometry of the molecule. All the aldo-hexoses form predominantly pyranose rings. Ribose, an aldo-pentose, is too small to form a stable pyranose; it forms the furanose ring shown above. Fructose, a keto-hexose, likes to form a furanose ring (N&C Fig. 7-7). Fructose can also cyclize to form pyranoses.
In the structures shown in Figure 13.19, the bonds to the anomeric OH groups are shown by a squiggly line pointing to the side. This notation is used when referring to either the alpha or the beta anomer, without specifying one or the other.
Stereochemistry of the ring forms: Haworth projections
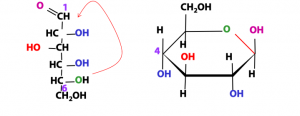
Dr. Norman Haworth drew the ring forms of sugars as shown in Figure 13.20. We call them “Haworth structures”. The second-last C atom in the Fischer formula (C-5) is the one that contributes the -OH group to form the hemiacetal; that’s the carbon atom at the top left of the ring, in the Haworth structure. The hemiacetal oxygen atom is in the ring, at the top right. The last C atom in the Fischer formula (C-6) is not chiral; it is CH2OH. That’s the one that is outside the ring, at the top. Each of the remaining C atoms (numbers 2, 3, and 4) has an -OH group either above or below the ring. If the -OH group points to the left in the Fischer formula, it goes above the ring in the Haworth formula, and vice versa. The ring forms of sugars have an additional chiral carbon: the anomeric carbon. If the configuration of the anomeric OH group is beta, it’s shown above the ring; if it is alpha it’s shown below the ring.
Note: The H atoms (C-H groups) are usually left out of Haworth structures for simplicity (although they are shown here); we are supposed to understand that they are there.
Reducing Sugars
LABORATORY-RELATED CONTENT
The information discussed in this section is related to Lab 4.
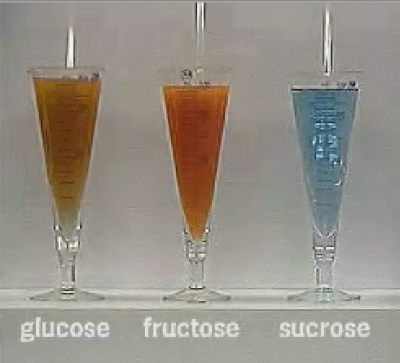
Some oxidants, such as cupric ion (Cu2+), can oxidize the carbonyl carbons of sugars to the corresponding acids. The Cu2+ gets reduced to cuprous ion (Cu+), which forms a red precipitate. Any sugar that reacts like this is called a “reducing sugar”. Some sugars don’t react with oxidants – they are “non-reducing sugars”. Sucrose (table sugar) is a “non-reducing” sugar: note that no red precipitate is formed in the glass container in Figure 13.21.
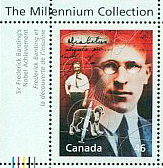
This chemical property provides a simple basis for detecting the presence and concentration of a sugar like glucose (e.g., Fehling’s reaction). It’s one of the ways that Dr. Frederic Banting measured blood glucose, back in the 1920s, when he was isolating insulin (Figure 13.22). (Nowadays, we have better methods for measuring blood glucose levels)
The anomeric carbon atom of a sugar is reactive
The anomeric carbon of the ring form of a sugar is the place where most of the chemical action occurs. Why? Well, the anomeric carbon is the only carbon atom that is bonded to two oxygen atoms. This makes the chemistry of the anomeric carbon special. It is electrophilic and reactive: For example, it can react with an alcohol.
Glycosides
As noted above, sugars, in their ring (hemiacetal or hemiketal) forms, have reactive anomeric carbon atoms. Nucleophiles can react at the anomeric carbon. The reaction is a condensation (formation of water), and a new bond is formed. The bond is a C-O bond for an alcohol and a C-N bond for an amine. We call the product a glycoside and we call the new bond a glycosidic (C-O) or a glycosylic (C-N) bond. Glycoside formation is the most important reaction of sugars.
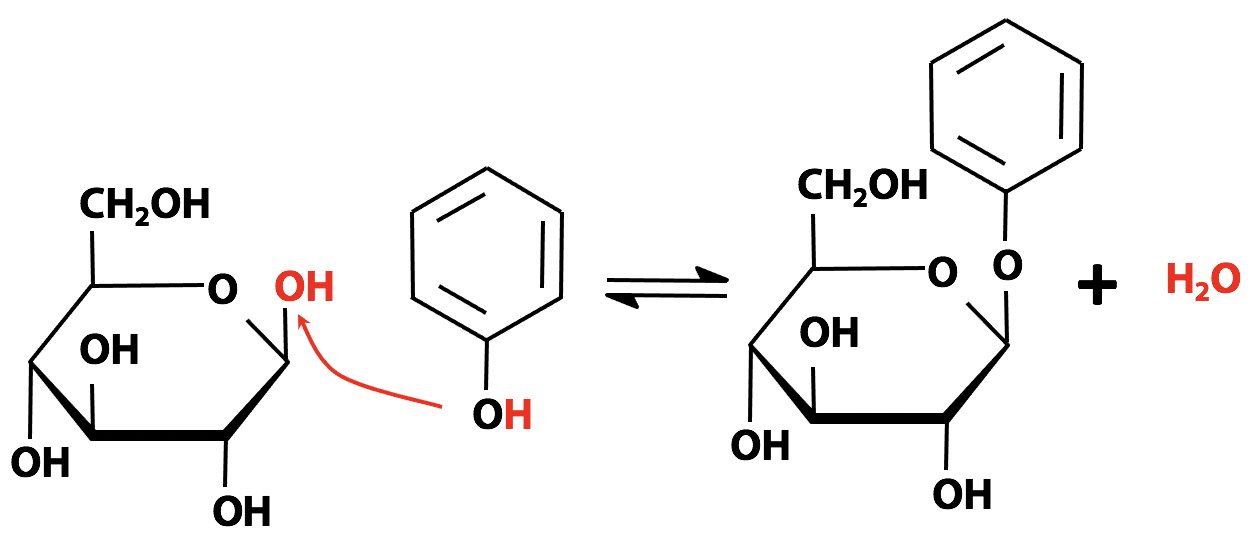
Figure 13.23 above shows a glycoside formed from phenol (C6H5OH) and glucose. Sugars are also alcohols, of course. In principle, any -OH group on a sugar can act as a nucleophile and form a glycosidic bond to the anomeric carbon atom of another sugar. This is how sugars are assembled into polymers (oligosaccharides) such as starch and cellulose. Later, we will see that nitrogenous bases can also form glycosides – that is how the nucleoside units of nucleic acids are put together. Lots of natural products obtained from plants are glycosides. For example, the foxglove plant produces a compound called digoxin (“digitalis”) (Figure 12.24), which is a valuable medicine for treating heart problems. Digitalis is a glycoside; when it is ingested, the glycosidic bond is broken by metabolism, releasing the active drug.
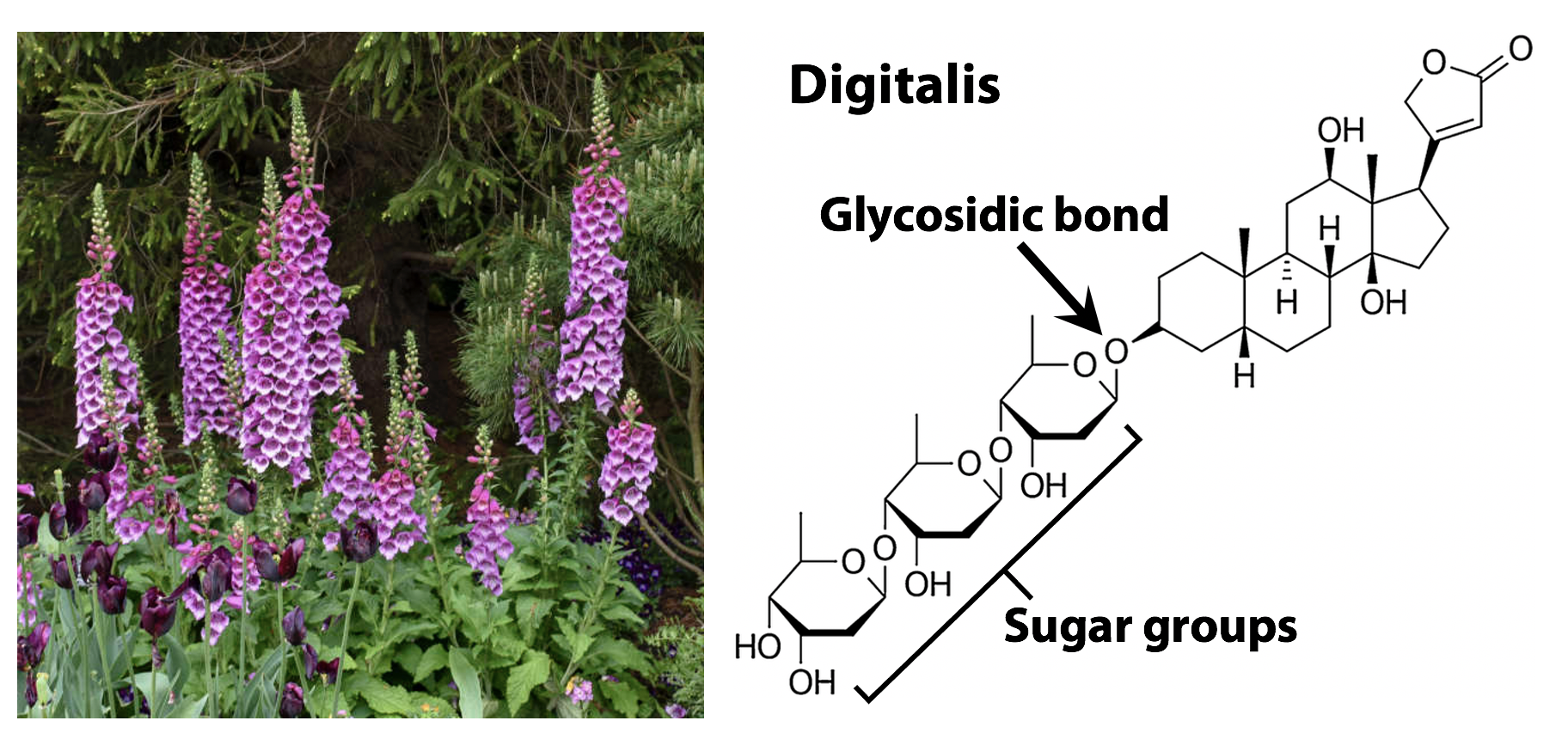
Non-reducing sugars
The oxidation of sugars by cupric ions (the reaction that defines a reducing sugar) only occurs with the open-chain aldehyde or ketone forms of sugars, which exist in equilibrium with the cyclic forms. In the glycoside, the anomeric hydroxyl group on the sugar is replaced by the glycosidic bond. To reverse the formation of a ring (that is, to return the sugar to a linear form – see “cyclization of glucose”), we would need to break the O-H bond of the anomeric -OH group. But we can’t, in a glycoside, because that H atom is gone. So, this glycoside is not able to open up into a linear form; cannot form an open-chain aldehyde or ketone; and, consequently, is called a non-reducing sugar.
Disaccharides
A disaccharide is built from two simple sugars, by condensation. In this reaction, the anomeric C of one sugar is the electrophile and any one of the hydroxyl (OH) groups of the second sugar is the nucleophile. Chemically speaking, the product is a glycoside, as we saw earlier; but we usually refer to it as a disaccharide, because both portions are sugars. Figure 13.25 shows the formation of a glycosidic bond between C4 of glucose and C1 (anomeric carbon) of galactose. The product is called lactose; that’s the main sugar in milk.
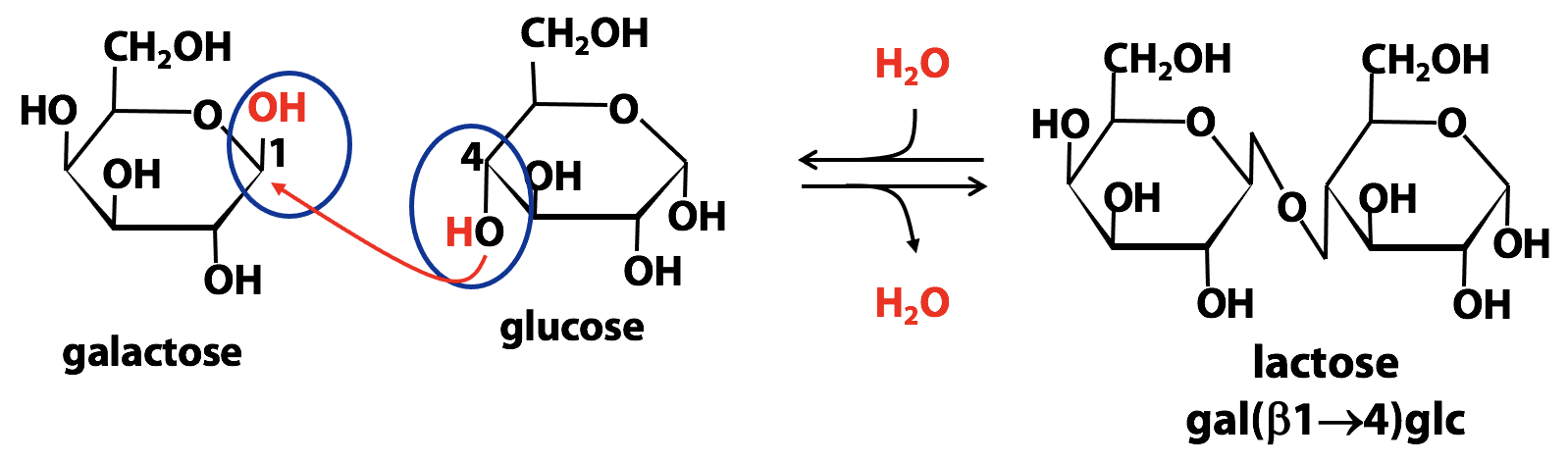
There are several tricky issues here, and we need to discuss them one by one:
Formation of the glycosidic bond of a disaccharide must involve the anomeric C of one sugar, because that is the only electrophilic C atom in a sugar. On the other hand, the OH group (the nucleophile) could be the OH on the anomeric C of a second sugar – or, as shown above, it could be any other -OH group in the sugar. So galactose and glucose could form lots of different disaccharides besides lactose; they would be structural isomers of lactose.
The orientation of the OH group on the anomeric carbon can be “up” (β) or “down” (α). So, the glycosidic bond from the anomeric carbon (C1 of galactose, in this example) could be either “up” or “down”. In the case of lactose, it is “up”. So the glycosidic bond is described as “β1→4”. This means that it goes from carbon-1 of galactose, in the β configuration, to the 4 carbon of glucose: lactose is “galactose (β1→4) glucose”, or “gal(β1→4)glu”.
Notice that the anomeric OH group of glucose is still free, in lactose. So the glucose part of this disaccharide can still open up into the linear form (open-chain aldehyde form). As a result, lactose is a reducing sugar. And, like any reducing sugar, it can undergo mutarotation, exist in either α or β anomeric forms. So, you can buy two kinds of lactose: α-lactose and β-lactose. In solution, they form an equilibrium mixture, just as glucose does. Note that the glycosidic bond in lactose is still β1→4, regardless of the anomeric form at glucose C1. In disaccharides and polysaccharides, the end of a chain with a free anomeric carbon (one not involved in a glycosidic bond) is called the reducing end.
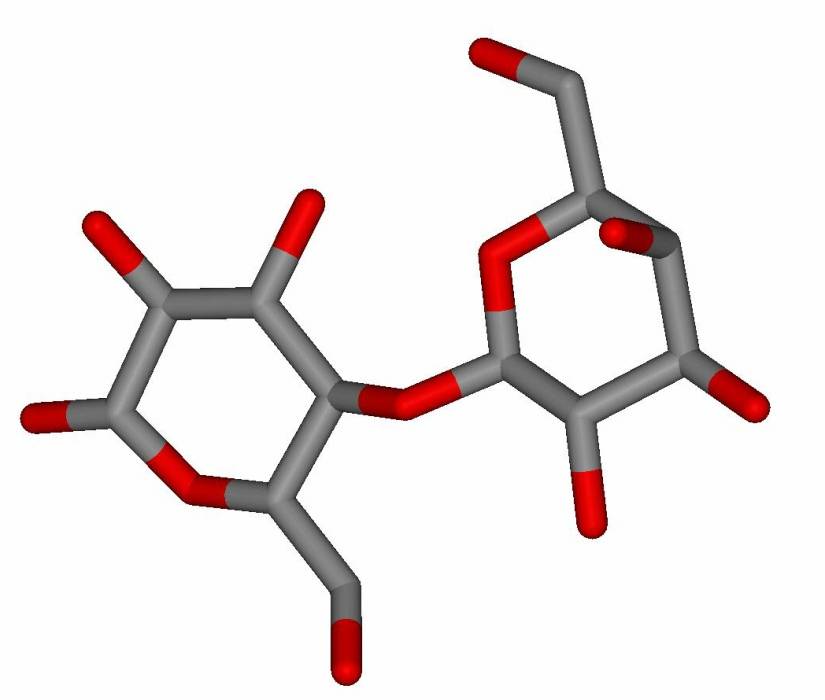
Could we form a non-reducing sugar from galactose and glucose? Yes. That would happen if a glycosidic bond were formed between the anomeric C of galactose and the anomeric OH group of glucose. It does not have an anomeric -OH group anymore.
A true-to-life image of lactose is shown in Figure 13.26. Notice that the glucose half of the molecule is flipped upside-down relative to the galactose half. (That’s because of the relative orientations of the two OH groups that reacted to form the glycosidic bond in lactose.) We usually draw both sugars the “usual” way, i.e., with C-6 at the top left. But then, to show the configuration of the glycosidic bond, we are forced to draw a zig-zag, or very long bonds, as shown in the drawing.
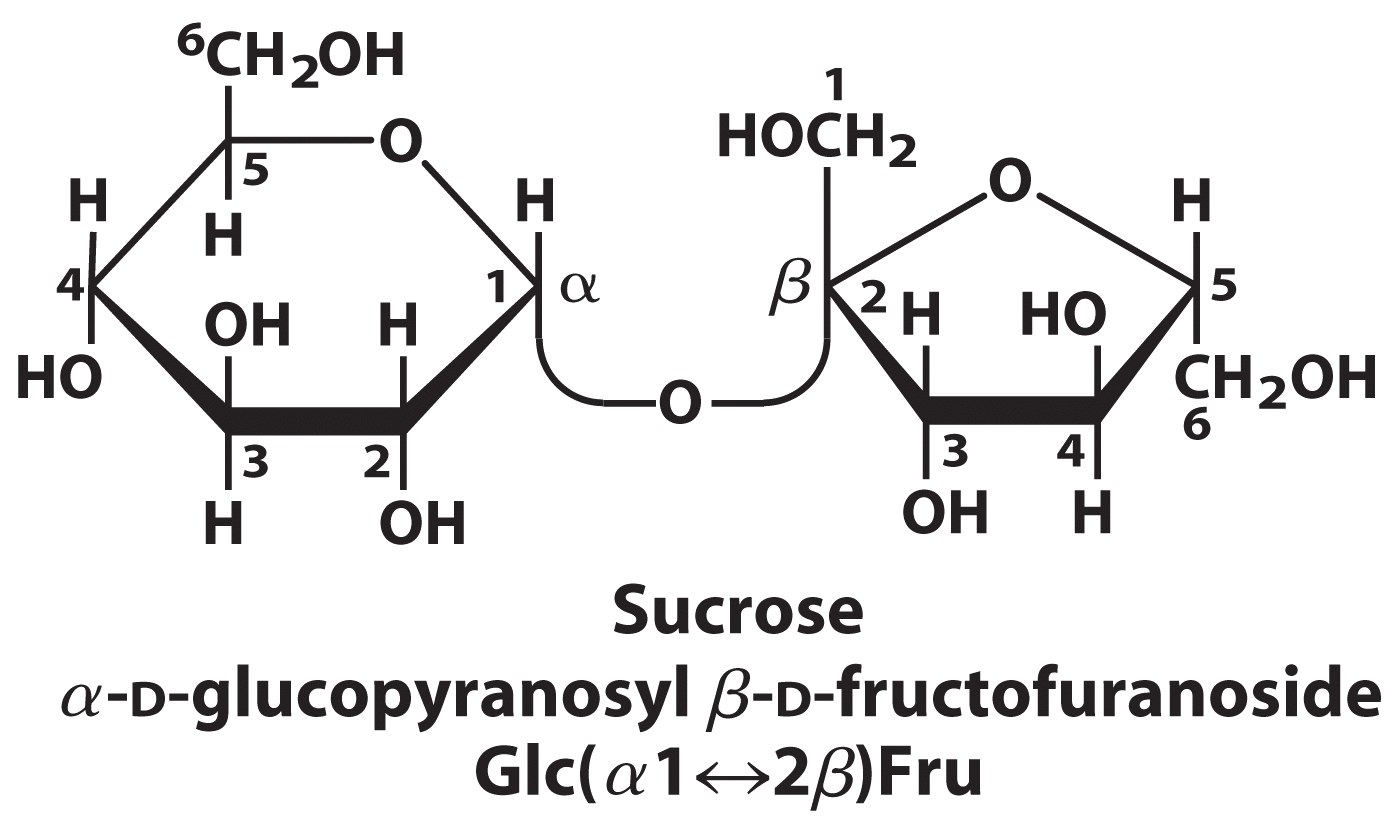
Sucrose (common table sugar), is a disaccharide formed from one unit of glucose and one unit of fructose. The anomeric carbons of both glucose and fructose are involved in the glycosidic bond; sucrose; therefore, is a non-reducing sugar. In Figure 13.27, the double headed arrow is used to show the involvement of both anomeric carbon atoms in the glycosidic linkage.
(The structure of sucrose, as shown, often causes confusion. The fructose half “looks wrong”, when compared to the Haworth structure of fructose shown in the Stryer textbook (Fig. 11.5). This is because the fructose half of the disaccharide has been “flipped over”, in drawing the sucrose structure. So the substituents that had been pointing “up” are now pointing “down”, and vice versa. To avoid this sort of confusion, it is better to draw the disaccharides with the sugars in their standard orientations, even at the cost of having to draw very long, looping glycosidic bonds.)
Maltose and trehalose are two of many possible glucose disaccharides. Maltose is a reducing sugar, while trehalose is a non-reducing sugar as it does not contain a free anomeric carbon atom. In the abbreviated nomenclature, a double headed arrow is used to show the involvement of both anomeric carbon atoms in the glycosidic linkage.
(You are not expected to memorize the structures of disaccharides; there are very many of them. But you should be able to look at a disaccharide and figure out how it is put together).
Polysaccharides
Most carbohydrates found in nature occur as polysaccharides; polymers of monosaccharide units. Unlike proteins, which are linear polymers, polysaccharides are often highly branched. Branching can happen – as we have seen – sugars have several OH groups, each of which can act as the nucleophile in forming a glycosidic bond. Therefore, a single sugar subunit can bond to two (or more) other subunits, making branches possible. (Branching can’t happen with amino acids, because each amino acid has only one amino group and one carboxylic acid group (unless side-chains start getting involved).
Polysaccharides differ from each other in the identity of the sugar units that are linked, in the length of the chains, in the type of bonds linking the units, and in the degree of branching.
If the polysaccharide is made up of a single type of sugar monomer, it’s a homopolysaccharide, such as a glucan or mannan. The glucose homopolymers, starch (plants) and glycogen (animals) serve as storage forms of glucose. Cellulose and chitin are homopolysaccharides that serve as structural elements in plant cell walls and animal exoskeletons. Heteropolysaccharides are those sugars built from two or more kinds of sugar subunit.