24 Seeing
Original chapter by Charles Stangor with adaptations by Jennifer Walinga, adapted by the Queen’s University Psychology Department
We encourage students to use the “Three-Step Method” for support in their learning. Please find our version of the Three-Step Method, created in collaboration with Queen’s Student Academic Success Services, at the following link: https://sass.queensu.ca/psyc100/
You’ll notice that this chapter looks a bit different from our earlier chapters. A benefit of an Open Access textbook is that we have the ability to source and adapt content written by experts globally that address issues that are important for our course. This chapter is from the text “Introduction to Psychology–1st Canadian Edition.” You can find the book here.
Learning Objectives
- Identify the key structures of the eye and the role they play in vision.
- Summarize how the eye and the visual cortex work together to sense and perceive the visual stimuli in the environment, including processing colours, shape, depth, and motion
Whereas other animals rely primarily on hearing, smell, or touch to understand the world around them, human beings rely in large part on vision. A large part of our cerebral cortex is devoted to seeing, and we have substantial visual skills. Seeing begins when light falls on the eyes, initiating the process of transduction. Once this visual information reaches the visual cortex, it is processed by a variety of neurons that detect colours, shapes, and motion, and that create meaningful perceptions out of the incoming stimuli.
The air around us is filled with a sea of electromagnetic energy: pulses of energy waves that can carry information from place to place. Electromagnetic waves vary in their wavelength— the distance between one wave peak and the next wave peak — with the shortest gamma waves being only a fraction of a millimeter in length and the longest radio waves being hundreds of kilometers long. Humans are blind to almost all of this energy — our eyes detect only the range from about 400 to 700 billionths of a meter, the part of the electromagnetic spectrum known as the visible spectrum.
The Sensing Eye and the Perceiving Visual Cortex
As you can see in Figure 5.7, “Anatomy of the Human Eye,” light enters the eye through the cornea, a clear covering that protects the eye and begins to focus the incoming light. The light then passes through the pupil, a small opening in the centre of the eye. The pupil is surrounded by the iris, the coloured part of the eye that controls the size of the pupil by constricting or dilating in response to light intensity. When we enter a dark movie theatre on a sunny day, for instance, muscles in the iris open the pupil and allow more light to enter. Complete adaptation to the dark may take up to 20 minutes.
Behind the pupil is the lens, a structure that focuses the incoming light on the retina, the layer of tissue at the back of the eye that contains photoreceptor cells. As our eyes move from near objects to distant objects, a process known as visual accommodation occurs. Visual accommodation is the process of changing the curvature of the lens to keep the light entering the eye focused on the retina. Rays from the top of the image strike the bottom of the retina and vice versa, and rays from the left side of the image strike the right part of the retina and vice versa, causing the image on the retina to be upside down and backward. Furthermore, the image projected on the retina is flat, and yet our final perception of the image will be three dimensional.
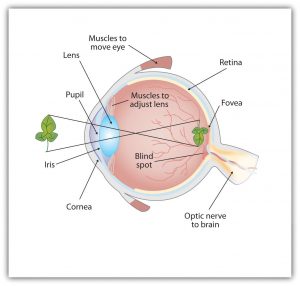
Accommodation is not always perfect (Figure 5.8) if the focus is in front of the retina, we say that the person is nearsighted, and when the focus is behind the retina, we say that the person is farsighted. Eyeglasses and contact lenses correct this problem by adding another lens in front of the eye, and laser eye surgery corrects the problem by reshaping the eye’s own lens.
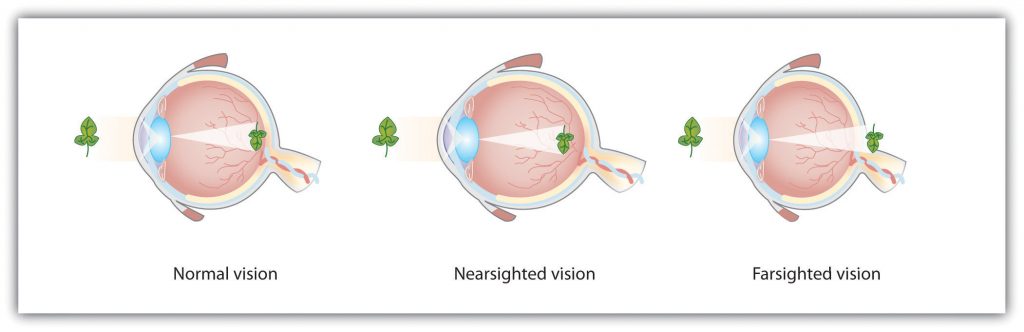
The retina contains layers of neurons specialized to respond to light (see Figure 5.9, “The Retina with Its Specialized Cells”). As light falls on the retina, it first activates receptor cells known as rods and cones. The activation of these cells then spreads to the bipolar cells and then to the ganglion cells, which gather together and converge, like the strands of a rope, forming the optic nerve. The optic nerve is a collection of millions of ganglion neurons that sends vast amounts of visual information, via the thalamus, to the brain. Because the retina and the optic nerve are active processors and analyzers of visual information, it is appropriate to think of these structures as an extension of the brain itself.
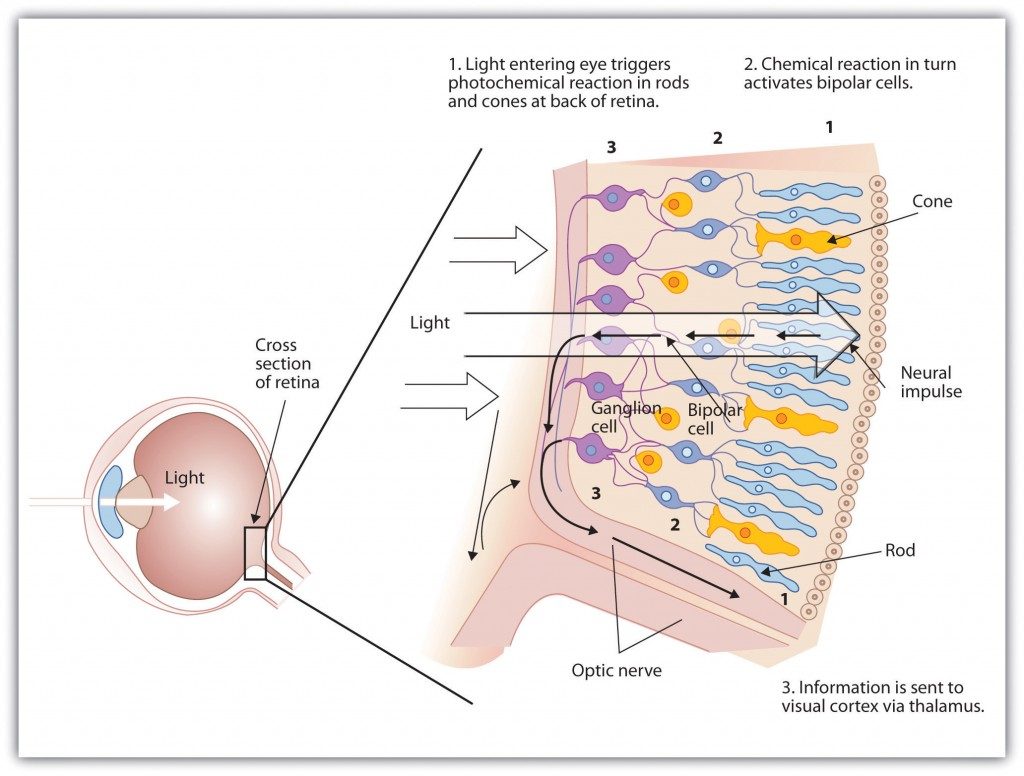
Rods are visual neurons that specialize in detecting black, white, and gray colours. There are about 120 million rods in each eye. The rods do not provide a lot of detail about the images we see, but because they are highly sensitive to shorter-waved (darker) and weak light, they help us see in dim light — for instance, at night. Because the rods are located primarily around the edges of the retina, they are particularly active in peripheral vision (when you need to see something at night, try looking away from what you want to see). Cones are visual neurons that are specialized in detecting fine detail and colours. The five million or so cones in each eye enable us to see in colour, but they operate best in bright light. The cones are located primarily in and around the fovea, which is the central point of the retina.
To demonstrate the difference between rods and cones in attention to detail, choose a word in this text and focus on it. Do you notice that the words a few inches to the side seem more blurred? This is because the word you are focusing on strikes the detail-oriented cones, while the words surrounding it strike the less-detail-oriented rods, which are located on the periphery.
Margaret Livingstone (2000) (Figure 5.10) found an interesting effect that demonstrates the different processing capacities of the eye’s rods and cones — namely, that the Mona Lisa’s smile, which is widely referred to as “elusive,” is perceived differently depending on how one looks at the painting. Because Leonardo da Vinci painted the smile in low-detail brush strokes, these details are better perceived by our peripheral vision (the rods) than by the cones. Livingstone found that people rated the Mona Lisa as more cheerful when they were instructed to focus on her eyes than they did when they were asked to look directly at her mouth. As Livingstone put it, “She smiles until you look at her mouth, and then it fades, like a dim star that disappears when you look directly at it.”
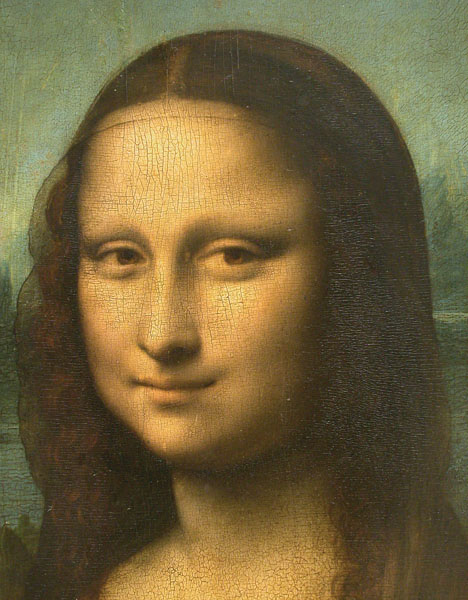
As you can see in Figure 5.11, “Pathway of Visual Images through the Thalamus and into the Visual Cortex,” the sensory information received by the retina is relayed through the thalamus to corresponding areas in the visual cortex, which is located in the occipital lobe at the back of the brain. Although the principle of contralateral control might lead you to expect that the left eye would send information to the right brain hemisphere and vice versa, nature is smarter than that. In fact, the left and right eyes each send information to both the left and the right hemisphere, and the visual cortex processes each of the cues separately and in parallel. This is an adaptational advantage to an organism that loses sight in one eye, because even if only one eye is functional, both hemispheres will still receive input from it.
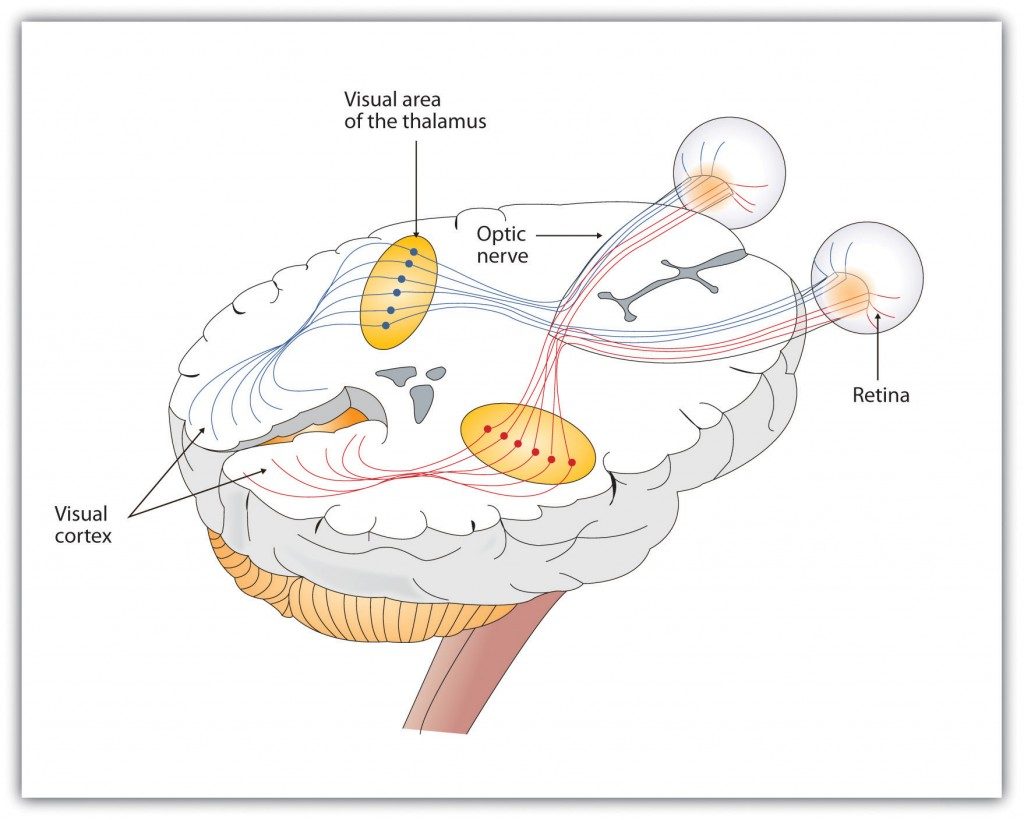
The visual cortex is made up of specialized neurons that turn the sensations they receive from the optic nerve into meaningful images. Because there are no photoreceptor cells at the place where the optic nerve leaves the retina, a hole or blind spot in our vision is created (see Figure 5.12, “Blind Spot Demonstration”). When both of our eyes are open, we don’t experience a problem because our eyes are constantly moving, and one eye makes up for what the other eye misses. But the visual system is also designed to deal with this problem if only one eye is open — the visual cortex simply fills in the small hole in our vision with similar patterns from the surrounding areas, and we never notice the difference. The ability of the visual system to cope with the blind spot is another example of how sensation and perception work together to create meaningful experience.
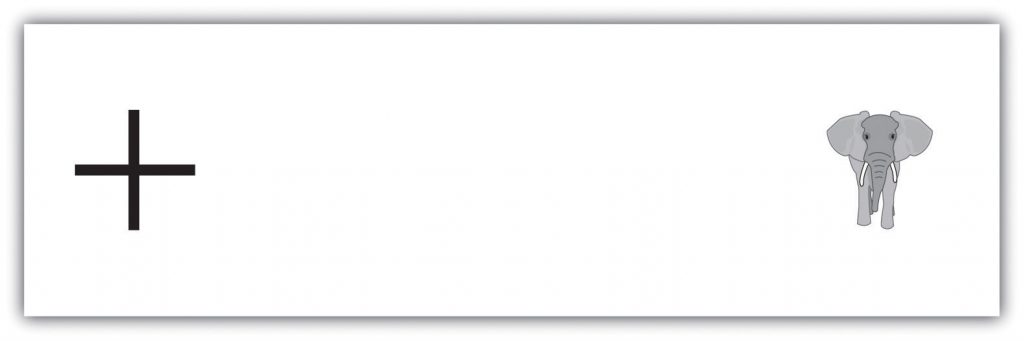
Perception is created in part through the simultaneous action of thousands of feature detector neurons — specialized neurons, located in the visual cortex, that respond to the strength, angles, shapes, edges, and movements of a visual stimulus (Kelsey, 1997; Livingstone & Hubel, 1988). The feature detectors work in parallel, each performing a specialized function. When faced with a red square, for instance, the parallel line feature detectors, the horizontal line feature detectors, and the red colour feature detectors all become activated. This activation is then passed on to other parts of the visual cortex, where other neurons compare the information supplied by the feature detectors with images stored in memory. Suddenly, in a flash of recognition, the many neurons fire together, creating the single image of the red square that we experience (Rodriguez et al., 1999). See Figure 5.13 for an explanation about the Necker cube.
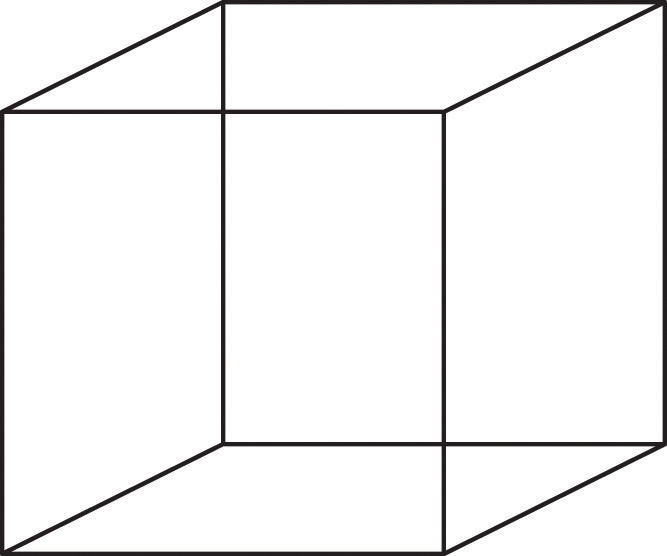
Some feature detectors are tuned to selectively respond to particularly important objects, such as faces, smiles, and other parts of the body (Downing, Jiang, Shuman, & Kanwisher, 2001; Haxby et al., 2001). When researchers disrupted face recognition areas of the cortex using the magnetic pulses of transcranial magnetic stimulation (TMS), people were temporarily unable to recognize faces, and yet they were still able to recognize houses (McKone, Kanwisher, & Duchaine, 2007; Pitcher, Walsh, Yovel, & Duchaine, 2007).
Perceiving Colour
It has been estimated that the human visual system can detect and discriminate among seven million colour variations (Geldard, 1972), but these variations are all created by the combinations of the three primary colours: red, green, and blue. The shade of a colour, known as hue, is conveyed by the wavelength of the light that enters the eye (we see shorter wavelengths as more blue and longer wavelengths as more red), and we detect brightness from the intensity or height of the wave (bigger or more intense waves are perceived as brighter).
In his important research on colour vision, Hermann von Helmholtz (1821-1894) theorized that colour is perceived because the cones in the retina come in three types. One type of cone reacts primarily to blue light (short wavelengths), another reacts primarily to green light (medium wavelengths), and a third reacts primarily to red light (long wavelengths). The visual cortex then detects and compares the strength of the signals from each of the three types of cones, creating the experience of colour. According to this Young-Helmholtz trichromatic colour theory what colour we see depends on the mix of the signals from the three types of cones. If the brain is receiving primarily red and blue signals, for instance, it will perceive purple; if it is receiving primarily red and green signals it will perceive yellow; and if it is receiving messages from all three types of cones it will perceive white.
The different functions of the three types of cones are apparent in people who experience colour blindness — the inability to detect green and/or red colours. About one in 50 people, mostly men, lack functioning in the red- or green-sensitive cones, leaving them only able to experience either one or two colours (Figure 5.15).
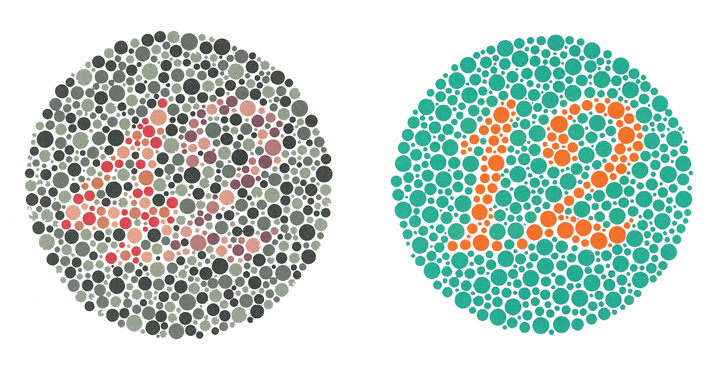
The trichromatic colour theory cannot explain all of human vision, however. For one, although the colour purple does appear to us as a mix of red and blue, yellow does not appear to be a mix of red and green. And people with colour blindness, who cannot see either green or red, nevertheless can still see yellow. An alternative approach to the Young-Helmholtz theory, known as the opponent-process colour theory, proposes that we analyze sensory information not in terms of three colours but rather in three sets of “opponent colours”: red-green, yellow-blue, and white-black. Evidence for the opponent-process theory comes from the fact that some neurons in the retina and in the visual cortex are excited by one colour (e.g., red) but inhibited by another colour (e.g., green).
One example of opponent processing occurs in the experience of an afterimage. If you stare at the shape on the top left side of Figure 5.16, “Afterimages,” for about 30 seconds (the longer you look, the better the effect), and then move your eyes to the blank area to the right of it, you will see the afterimage. Now try this by staring at the image of the Italian flag below and then shifting your eyes to the blank area beside it. When we stare at the green stripe, our green receptors habituate and begin to process less strongly, whereas the red receptors remain at full strength. When we switch our gaze, we see primarily the red part of the opponent process. Similar processes create blue after yellow and white after black.
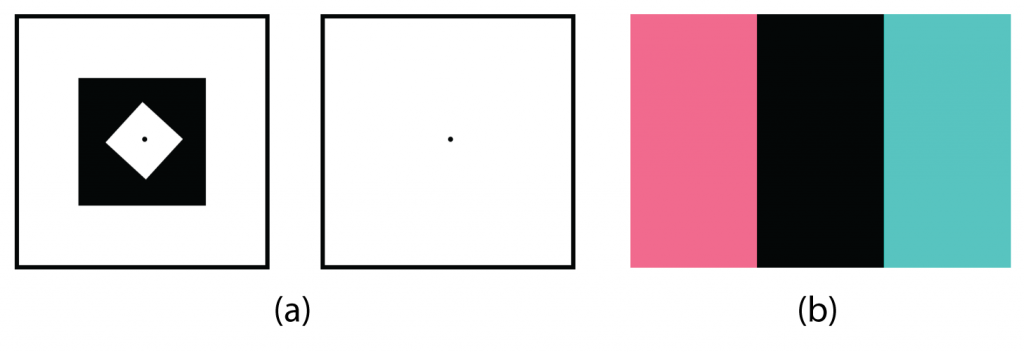
The tricolour and the opponent-process mechanisms work together to produce colour vision. When light rays enter the eye, the red, blue, and green cones on the retina respond in different degrees and send different strength signals of red, blue, and green through the optic nerve. The colour signals are then processed both by the ganglion cells and by the neurons in the visual cortex (Gegenfurtner & Kiper, 2003).
Perceiving Form
One of the important processes required in vision is the perception of form. German psychologists in the 1930s and 1940s, including Max Wertheimer (1880-1943), Kurt Koffka (1886-1941), and Wolfgang Köhler (1887-1967), argued that we create forms out of their component sensations based on the idea of the gestalt, a meaningfully organized whole. The idea of the gestalt is that the “whole is more than the sum of its parts.” Some examples of how gestalt principles lead us to see more than what is actually there are summarized in Table 5.1, “Summary of Gestalt Principles of Form Perception.”
Table 5.1 Summary of Gestalt Principles of Form Perception.
Table 5.1 Summary of Gestalt Principles of Form Perception.
Depth perception is the ability to perceive three-dimensional space and to accurately judge distance. Without depth perception, we would be unable to drive a car, thread a needle, or simply navigate our way around the supermarket (Howard & Rogers, 2001). Research has found that depth perception is in part based on innate capacities and in part learned through experience (Witherington, 2005).
Perceiving Depth
Psychologists Eleanor Gibson and Richard Walk (1960) tested the ability to perceive depth in six- to 14-month-old infants by placing them on a visual cliff, a mechanism that gives the perception of a dangerous drop-off, in which infants can be safely tested for their perception of depth (Figure 5.17 “Visual Cliff”). The infants were placed on one side of the “cliff,” while their mothers called to them from the other side. Gibson and Walk found that most infants either crawled away from the cliff or remained on the board and cried because they wanted to go to their mothers, but the infants perceived a chasm that they instinctively could not cross. Further research has found that even very young children who cannot yet crawl are fearful of heights (Campos, Langer, & Krowitz, 1970). On the other hand, studies have also found that infants improve their hand-eye coordination as they learn to better grasp objects and as they gain more experience in crawling, indicating that depth perception is also learned (Adolph, 2000).
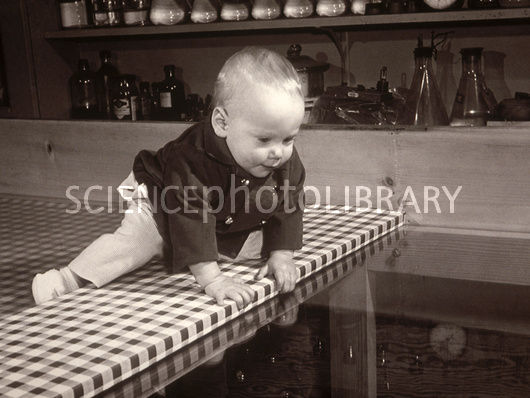
Depth perception is the result of our use of depth cues, messages from our bodies and the external environment that supply us with information about space and distance. Binocular depth cues are depth cues that are created by retinal image disparity — that is, the space between our eyes — and which thus require the coordination of both eyes. One outcome of retinal disparity is that the images projected on each eye are slightly different from each other. The visual cortex automatically merges the two images into one, enabling us to perceive depth. Three-dimensional movies make use of retinal disparity by using 3-D glasses that the viewer wears to create a different image on each eye. The perceptual system quickly, easily, and unconsciously turns the disparity into 3-D.
An important binocular depth cue is convergence, the inward turning of our eyes that is required to focus on objects that are less than about 50 feet away from us. The visual cortex uses the size of the convergence angle between the eyes to judge the object’s distance. You will be able to feel your eyes converging if you slowly bring a finger closer to your nose while continuing to focus on it. When you close one eye, you no longer feel the tension — convergence is a binocular depth cue that requires both eyes to work.
The visual system also uses accommodation to help determine depth. As the lens changes its curvature to focus on distant or close objects, information relayed from the muscles attached to the lens helps us determine an object’s distance. Accommodation is only effective at short viewing distances, however, so while it comes in handy when threading a needle or tying shoelaces, it is far less effective when driving or playing sports.
Although the best cues to depth occur when both eyes work together, we are able to see depth even with one eye closed. Monocular depth cues are depth cues that help us perceive depth using only one eye (Sekuler & Blake, 2006). Some of the most important are summarized in Table 5.2, “Monocular Depth Cues That Help Us Judge Depth at a Distance.”
Perceiving Motion
Many animals, including human beings, have very sophisticated perceptual skills that allow them to coordinate their own motion with the motion of moving objects in order to create a collision with that object. Bats and birds use this mechanism to catch up with prey, dogs use it to catch a Frisbee, and humans use it to catch a moving football. The brain detects motion partly from the changing size of an image on the retina (objects that look bigger are usually closer to us) and in part from the relative brightness of objects.
We also experience motion when objects near each other change their appearance. The beta effect refers to the perception of motion that occurs when different images are presented next to each other in succession (see “Beta Effect and Phi Phenomenon”). The visual cortex fills in the missing part of the motion and we see the object moving. The beta effect is used in movies to create the experience of motion. A related effect is the phi phenomenon, in which we perceive a sensation of motion caused by the appearance and disappearance of objects that are near each other. The phi phenomenon looks like a moving zone or cloud of background colour surrounding the flashing objects. The beta effect and the phi phenomenon are other examples of the importance of the gestalt — our tendency to “see more than the sum of the parts.”
Beta Effect and Phi Phenomenon
In the beta effect, our eyes detect motion from a series of still images, each with the object in a different place. This is the fundamental mechanism of motion pictures (movies). In the phi phenomenon, the perception of motion is based on the momentary hiding of an image.
Phi phenomenon: http://upload.wikimedia.org/wikipedia/commons/6/6e/Lilac-Chaser.gif
Beta effect: http://upload.wikimedia.org/wikipedia/commons/0/09/Phi_phenomenom_no_watermark.gif
Key Takeaways
- Vision is the process of detecting the electromagnetic energy that surrounds us. Only a small fraction of the electromagnetic spectrum is visible to humans.
- The visual receptor cells on the retina detect shape, colour, motion, and depth.
- Light enters the eye through the transparent cornea and passes through the pupil at the centre of the iris. The lens adjusts to focus the light on the retina, where it appears upside down and backward. Receptor cells on the retina are excited or inhibited by the light and send information to the visual cortex through the optic nerve.
- The retina has two types of photoreceptor cells: rods, which detect brightness and respond to black and white, and cones, which respond to red, green, and blue. Colour blindness occurs when people lack function in the red- or green-sensitive cones.
- Feature detector neurons in the visual cortex help us recognize objects, and some neurons respond selectively to faces and other body parts.
- The Young-Helmholtz trichromatic colour theory proposes that colour perception is the result of the signals sent by the three types of cones, whereas the opponent-process colour theory proposes that we perceive colour as three sets of opponent colours: red-green, yellow-blue, and white-black.
- The ability to perceive depth occurs as the result of binocular and monocular depth cues.
- Motion is perceived as a function of the size and brightness of objects. The beta effect and the phi phenomenon are examples of perceived motion.
Check Your Knowledge
To help you with your studying, we’ve included some practice questions for this module. These questions do not necessarily address all content in this module. They are intended as practice, and you are responsible for all of the content in this module even if there is no associated practice question. To promote deeper engagement with the material, we encourage you to create some questions of your own for your practice. You can then also return to these self-generated questions later in the course to test yourself.
Vocabulary
- Accommodation
- Helps determine depth.
- Beta effect
- The perception of motion that occurs when different images are presented next to each other in succession
- Binocular depth cues
- Depth cues that are created by retinal image disparity — that is, the space between our eyes — and which thus require the coordination of both eyes
- Blind spot
- A hole in our vision because there are no photoreceptor cells at the place where the optic nerve leaves the retina
- Colour blindness
- The inability to detect green and/or red colours
- Cones
- Visual neurons that are specialized in detecting fine detail and colours
- Convergence
- The inward turning of our eyes that is required to focus on objects that are less than about 50 feet away from us
- Cornea
- A clear covering that protects the eye and begins to focus the incoming light
- Depth cues
- Messages from our bodies and the external environment that supply us with information about space and distance.
- Electromagnetic energy
- Pulses of energy waves that can carry information from place to place
- Farsighted
- When the focus is behind the retina
- Feature detector neurons
- Specialized neurons, located in the visual cortex, that respond to the strength, angles, shapes, edges, and movements of a visual stimulus
- Fovea
- The central point of the retina
- Gestalt
- A meaningfully organized whole
- Hue
- The shade of a colour
- Iris
- The coloured part of the eye that controls the size of the pupil by constricting or dilating in response to light intensity
- Lens
- A structure that focuses the incoming light on the retina
- Monocular depth cues
- Depth cues that help us perceive depth using only one eye
- Nearsighted
- When the focus is in front of the retina
- Opponent-process colour theory
- Proposes that we analyze sensory information not in terms of three colours but rather in three sets of “opponent colours”: red-green, yellow-blue, and white-black
- Optic nerve
- A collection of millions of ganglion neurons that sends vast amounts of visual information, via the thalamus, to the brain
- Phi phenomenon
- We perceive a sensation of motion caused by the appearance and disappearance of objects that are near each other
- Pupil
- A small opening in the centre of the eye
- Retina
- The layer of tissue at the back of the eye that contains photoreceptor cells
- Rods
- Visual neurons that specialize in detecting black, white, and gray colours
- Trichromatic colour theory
- The colour we see depends on the mix of the signals from the three types of cones
- Visible spectrum
- The part of the electromagnetic spectrum that our eyes detect (only the range from about 400 to 700 billionths of a meter)
- Visual accommodation
- The process of changing the curvature of the lens to keep the light entering the eye focused on the retina
- Visual cliff
- A mechanism that gives the perception of a dangerous drop-off, in which infants can be safely tested for their perception of depth
- Wavelength
- The distance between one wave peak and the next wave peak
References
Adolph, K. E. (2000). Specificity of learning: Why infants fall over a veritable cliff. Psychological Science, 11(4), 290–295.
Campos, J. J., Langer, A., & Krowitz, A. (1970). Cardiac responses on the visual cliff in prelocomotor human infants. Science, 170(3954), 196–197.
Downing, P. E., Jiang, Y., Shuman, M., & Kanwisher, N. (2001). A cortical area selective for visual processing of the human body. Science, 293(5539), 2470–2473.
Gegenfurtner, K. R., & Kiper, D. C. (2003). Color vision. Annual Review of Neuroscience, 26, 181–206.
Geldard, F. A. (1972). The human senses (2nd ed.). New York, NY: John Wiley & Sons.
Gibson, E. J., & Walk, R. D. (1960). The “visual cliff.” Scientific American, 202(4), 64–71.
Haxby, J. V., Gobbini, M. I., Furey, M. L., Ishai, A., Schouten, J. L., & Pietrini, P. (2001). Distributed and overlapping representations of faces and objects in ventral temporal cortex. Science, 293(5539), 2425–2430.
Howard, I. P., & Rogers, B. J. (2001). Seeing in depth: Basic mechanisms (Vol. 1). Toronto, ON: Porteous.
Kelsey, C.A. (1997). Detection of visual information. In W. R. Hendee & P. N. T. Wells (Eds.), The perception of visual information (2nd ed.). New York, NY: Springer Verlag.
Livingstone, M., & Hubel, D. (1998). Segregation of form, color, movement, and depth: Anatomy, physiology, and perception. Science, 240, 740–749.
Livingstone M. S. (2000). Is it warm? Is it real? Or just low spatial frequency? Science, 290, 1299.
McKone, E., Kanwisher, N., & Duchaine, B. C. (2007). Can generic expertise explain special processing for faces? Trends in Cognitive Sciences, 11, 8–15.
Pitcher, D., Walsh, V., Yovel, G., & Duchaine, B. (2007). TMS evidence for the involvement of the right occipital face area in early face processing. Current Biology, 17, 1568–1573.
Rodriguez, E., George, N., Lachaux, J.-P., Martinerie, J., Renault, B., & Varela, F. J. (1999). Perception’s shadow: Long-distance synchronization of human brain activity. Nature, 397(6718), 430–433.
Sekuler, R., & Blake, R. (2006). Perception (5th ed.). New York, NY: McGraw-Hill.
Witherington, D. C. (2005). The development of prospective grasping control between 5 and 7 months: A longitudinal study. Infancy, 7(2), 143–161.
Image Attributions
Figure 5.10: Mona Lisa detail face(http://commons.wikimedia.org/wiki/File:Mona_Lisa_detail_face.jpg) is in the public domain.
Figure 5.15: Ishihara Plate No.11(http://commons.wikimedia.org/wiki/File:Ishihara_11.PNG) and Ishihara Plate No.23 (http://commons.wikimedia.org/wiki/File:Ishihara_23.PNG) is in the public domain.
Figure 5.16: Nachbild by Freddy2001 (http://commons.wikimedia.org/wiki/File:Nachbild-1.svg) and Italian Flag Inverted by Pcessna (http://commons.wikimedia.org/wiki/File:ItalianFlagInverted.gif) is in the public domain.
Figure 5.17: Perception-Conception (http://perception-connection.wikispaces.com/3)+Key+Findings) used with CC-BY-SA 3.0 (http://creativecommons.org/licenses/by-sa/3.0/).
This course makes use of Open Educational Resources. Information on the original source of this chapter can be found below.
Introduction to Psychology: 1st Canadian Edition was adapted by Jennifer Walinga from Charles Stangor’s textbook, Introduction to Psychology. For information about what was changed in this adaptation, refer to the Copyright statement at the bottom of the home page. This adaptation is a part of the B.C. Open Textbook Project.
In October 2012, the B.C. Ministry of Advanced Education announced its support for the creation of open textbooks for the 40 highest-enrolled first and second year subject areas in the province’s public post-secondary system.
Open textbooks are open educational resources (OER); they are instructional resources created and shared in ways so that more people have access to them. This is a different model than traditionally copyrighted materials. OER are defined as “teaching, learning, and research resources that reside in the public domain or have been released under an intellectual property license that permits their free use and re-purposing by others.” (Hewlett Foundation).
BCcampus’ open textbooks are openly licensed using a Creative Commons license, and are offered in various e-book formats free of charge, or as printed books that are available at cost.
For more information about this project, please contact opentext@bccampus.ca.
If you are an instructor who is using this book for a course, please fill out an adoption form.
How to cite this Chapter using APA Style:
Seeing. (2019). Adapted for use by Queen’s University. Original chapter in C. Stangor and J. Walinga (Eds.), Introduction to Psychology: 1st Canadian Edition. BCcampus. Retrieved from https://opentextbc.ca/introductiontopsychology/.
Copyright and Acknowledgements
This material is licensed under a Creative Commons Attribution-NonCommercial-ShareAlike 4.0 International License, except where otherwise noted.
Pulses of energy waves that can carry information from place to place
The distance between one wave peak and the next wave peak
The part of the electromagnetic spectrum that our eyes detect (only the range from about 400 to 700 billionths of a meter)
A clear covering that protects the eye and begins to focus the incoming light
A small opening in the centre of the eye
The coloured part of the eye that controls the size of the pupil by constricting or dilating in response to light intensity
A structure that focuses the incoming light on the retina
Cell layer in the back of the eye containing photoreceptors.
The process of changing the curvature of the lens to keep the light entering the eye focused on the retina
When the focus is in front of the retina
When the focus is behind the retina
A collection of millions of ganglion neurons that sends vast amounts of visual information, via the thalamus, to the brain
Photoreceptors of the retina sensitive to low levels of light. Located around the fovea.
Photoreceptors of the retina sensitive to color. Located primarily in the fovea.
The central point of the retina
Livingstone M. S. (2000). Is it warm? Is it real? Or just low spatial frequency? Science, 290, 1299.
A hole in our vision that is created because there are no photoreceptor cells at the place where the optic nerve leaves the retina
Specialized neurons, located in the visual cortex, that respond to the strength, angles, shapes, edges, and movements of a visual stimulus
Kelsey, C.A. (1997). Detection of visual information. In W. R. Hendee & P. N. T. Wells (Eds.), The perception of visual information (2nd ed.). New York, NY: Springer Verlag.
Livingstone, M., & Hubel, D. (1998). Segregation of form, color, movement, and depth: Anatomy, physiology, and perception. Science, 240, 740–749.
Rodriguez, E., George, N., Lachaux, J.-P., Martinerie, J., Renault, B., & Varela, F. J. (1999). Perception’s shadow: Long-distance synchronization of human brain activity. Nature, 397(6718), 430–433.
Downing, P. E., Jiang, Y., Shuman, M., & Kanwisher, N. (2001). A cortical area selective for visual processing of the human body. Science, 293(5539), 2470–2473.
McKone, E., Kanwisher, N., & Duchaine, B. C. (2007). Can generic expertise explain special processing for faces? Trends in Cognitive Sciences, 11, 8–15.
Geldard, F. A. (1972). The human senses (2nd ed.). New York, NY: John Wiley & Sons.
The shade of a colour
The colour we see depends on the mix of the signals from the three types of cones
The inability to detect green and/or red colours
Proposes that we analyze sensory information not in terms of three colours but rather in three sets of “opponent colours”: red-green, yellow-blue, and white-black
Gegenfurtner, K. R., & Kiper, D. C. (2003). Color vision. Annual Review of Neuroscience, 26, 181–206.
A meaningfully organized whole
Howard, I. P., & Rogers, B. J. (2001). Seeing in depth: Basic mechanisms (Vol. 1). Toronto, ON: Porteous.
Witherington, D. C. (2005). The development of prospective grasping control between 5 and 7 months: A longitudinal study. Infancy, 7(2), 143–161.
Gibson, E. J., & Walk, R. D. (1960). The “visual cliff.” Scientific American, 202(4), 64–71.
A mechanism that gives the perception of a dangerous drop-off, in which infants can be safely tested for their perception of depth
Campos, J. J., Langer, A., & Krowitz, A. (1970). Cardiac responses on the visual cliff in prelocomotor human infants. Science, 170(3954), 196–197.
Adolph, K. E. (2000). Specificity of learning: Why infants fall over a veritable cliff. Psychological Science, 11(4), 290–295.
Messages from our bodies and the external environment that supply us with information about space and distance
Depth cues that are created by retinal image disparity — that is, the space between our eyes — and which thus require the coordination of both eyes
The inward turning of our eyes that is required to focus on objects that are less than about 50 feet away from us
Helps determine depth
Depth cues that help us perceive depth using only one eye
Sekuler, R., & Blake, R. (2006). Perception (5th ed.). New York, NY: McGraw-Hill.
The perception of motion that occurs when different images are presented next to each other in succession
We perceive a sensation of motion caused by the appearance and disappearance of objects that are near each other