4 Epigenetics in Psychology
Original chapter by Ian Weaver adapted by Queen’s University Psychology Department
This Open Access chapter was originally written for the NOBA project. Information on the NOBA project can be found below.
Early life experiences exert a profound and long-lasting influence on physical and mental health throughout life. The efforts to identify the primary causes of this have significantly benefited from studies of the epigenome—a dynamic layer of information associated with DNA that differs between individuals and can be altered through various experiences and environments. The epigenome has been heralded as a key “missing piece” of the etiological puzzle for understanding how development of psychological disorders may be influenced by the surrounding environment, in concordance with the genome. Understanding the mechanisms involved in the initiation, maintenance, and heritability of epigenetic states is thus an important aspect of research in current biology, particularly in the study of learning and memory, emotion, and social behavior in humans. Moreover, epigenetics in psychology provides a framework for understanding how the expression of genes is influenced by experiences and the environment to produce individual differences in behavior, cognition, personality, and mental health. In this module, we survey recent developments revealing epigenetic aspects of mental health and review some of the challenges of epigenetic approaches in psychology to help explain how nurture shapes nature.
Learning Objectives
- Explain what the term epigenetics means and the molecular machinery involved.
- Name and discuss important neural and developmental pathways that are regulated by epigenetic factors, and provide examples of epigenetic effects on personality traits and cognitive behavior.
- Understand how misregulation of epigenetic mechanisms can lead to disease states, and be able to discuss examples.
- Recognize how epigenetic machinery can be targets for therapeutic agents, and discuss examples.
Introduction
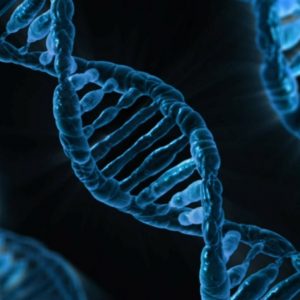
Early childhood is not only a period of physical growth; it is also a time of mental development related to changes in the anatomy, physiology, and chemistry of the nervous system that influence mental health throughout life. Cognitive abilities associated with learning and memory, reasoning, problem solving, and developing relationships continue to emerge during childhood. Brain development is more rapid during this critical or sensitive period than at any other, with more than 700 neural connections created each second. Herein, complex gene–environment interactions (or genotype–environment interactions, G×E) serve to increase the number of possible contacts between neurons, as they hone their adult synaptic properties and excitability. Many weak connections form to different neuronal targets; subsequently, they undergo remodeling in which most connections vanish and a few stable connections remain. These structural changes (or plasticity) may be crucial for the development of mature neural networks that support emotional, cognitive, and social behavior. The generation of different morphology, physiology, and behavioral outcomes from a single genome in response to changes in the environment forms the basis for “phenotypic plasticity,” which is fundamental to the way organisms cope with environmental variation, navigate the present world, and solve future problems.
The challenge for psychology has been to integrate findings from genetics and environmental (social, biological, chemical) factors, including the quality of infant–mother attachments, into the study of personality and our understanding of the emergence of mental illness. These studies have demonstrated that common DNA sequence variation and rare mutations account for only a small fraction (1%–2%) of the total risk for inheritance of personality traits and mental disorders (Dick, Riley, & Kendler, 2010; Gershon, Alliey-Rodriguez, & Liu, 2011). Additionally, studies that have attempted to examine the mechanisms and conditions under which DNA sequence variation influences brain development and function have been confounded by complex cause-and-effect relationships (Petronis, 2010). The large unaccounted heritability of personality traits and mental health suggests that additional molecular and cellular mechanisms are involved.
Epigenetics has the potential to provide answers to these important questions and refers to the transmission of phenotype in terms of gene expression in the absence of changes in DNA sequence—hence the name epi- (Greek: επί- over, above) genetics (Waddington, 1942; Wolffe & Matzke, 1999). The advent of high-throughput techniques such as sequencing-based approaches to study the distributions of regulators of gene expression throughout the genome led to the collective description of the “epigenome.” In contrast to the genome sequence, which is static and the same in almost all cells, the epigenome is highly dynamic, differing among cell types, tissues, and brain regions (Gregg et al., 2010). Recent studies have provided insights into epigenetic regulation of developmental pathways in response to a range of external environmental factors (Dolinoy, Weidman, & Jirtle, 2007). These environmental factors during early childhood and adolescence can cause changes in expression of genes conferring risk of mental health and chronic physical conditions. Thus, the examination of genetic–epigenetic–environment interactions from a developmental perspective may determine the nature of gene misregulation in psychological disorders.
This module will provide an overview of the main components of the epigenome and review themes in recent epigenetic research that have relevance for psychology, to form the biological basis for the interplay between environmental signals and the genome in the regulation of individual differences in physiology, emotion, cognition, and behavior.
Molecular control of gene expression: the dynamic epigenome
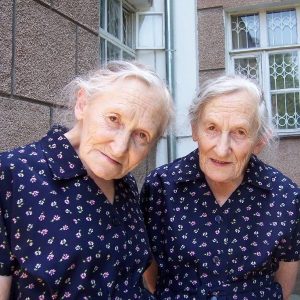
Almost all the cells in our body are genetically identical, yet our body generates many different cell types, organized into different tissues and organs, and expresses different proteins. Within each type of mammalian cell, about 2 meters of genomic DNA is divided into nuclear chromosomes. Yet the nucleus of a human cell, which contains the chromosomes, is only about 2 μm in diameter. To achieve this 1,000,000-fold compaction, DNA is wrapped around a group of 8 proteins called histones. This combination of DNA and histone proteins forms a special structure called a “nucleosome,” the basic unit of chromatin, which represents a structural solution for maintaining and accessing the tightly compacted genome. These factors alter the likelihood that a gene will be expressed or silenced. Cellular functions such as gene expression, DNA replication, and the generation of specific cell types are therefore influenced by distinct patterns of chromatin structure, involving covalent modification of both histones (Kadonaga, 1998) and DNA (Razin, 1998).
Importantly, epigenetic variation also emerges across the lifespan. For example, although identical twins share a common genotype and are genetically identical and epigenetically similar when they are young, as they age they become more dissimilar in their epigenetic patterns and often display behavioral, personality, or even physical differences, and have different risk levels for serious illness. Thus, understanding the structure of the nucleosome is key to understanding the precise and stable control of gene expression and regulation, providing a molecular interface between genes and environmentally induced changes in cellular activity.
The primary epigenetic mark: DNA modification
DNA methylation is the best-understood epigenetic modification influencing gene expression. DNA is composed of four types of naturally occurring nitrogenous bases: adenine (A), thymine (T), guanine (G), and cytosine (C). In mammalian genomes, DNA methylation occurs primarily at cytosine residues in the context of cytosines that are followed by guanines (CpG dinucleotides), to form 5-methylcytosine in a cell-specific pattern (Goll & Bestor, 2005; Law & Jacobsen, 2010; Suzuki & Bird, 2008). The enzymes that perform DNA methylation are called DNA methyltransferases (DNMTs), which catalyze the transfer of a methyl group to the cytosine (Adams, McKay, Craig, & Burdon, 1979). These enzymes are all expressed in the central nervous system and are dynamically regulated during development (Feng, Chang, Li, & Fan, 2005; Goto et al., 1994). The effect of DNA methylation on gene function varies depending on the period of development during which the methylation occurs and location of the methylated cytosine. Methylation of DNA in gene regulatory regions (promoter and enhancer regions) usually results in gene silencing and reduced gene expression (Ooi, O’Donnell, & Bestor, 2009; Suzuki & Bird, 2008; Sutter and Doerfler, 1980; Vardimon et al., 1982). This is a powerful regulatory mechanism that ensures that genes are expressed only when needed. Thus DNA methylation may broadly impact human brain development, and age-related misregulation of DNA methylation is associated with the molecular pathogenesis of neurodevelopmental disorders.
Histone modification and the histone code
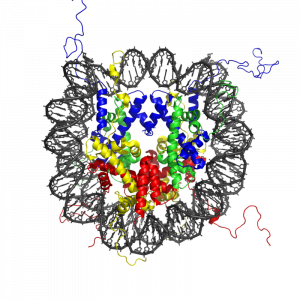
The modification of histone proteins comprises an important epigenetic mark related to gene expression. One of the most thoroughly studied modifications is histone acetylation, which is associated with gene activation and increased gene expression (Wade, Pruss, & Wolffe, 1997). Acetylation on histone tails is mediated by the opposing enzymatic activities of histone acetyltransferases (HATs) and histone deacetylases (HDACs) (Kuo & Allis, 1998). For example, acetylation of histone in gene regulatory regions by HAT enzymes is generally associated with DNA demethylation, gene activation, and increased gene expression (Hong, Schroth, Matthews, Yau, & Bradbury, 1993; Sealy & Chalkley, 1978). On the other hand, removal of the acetyl group (deacetylation) by HDAC enzymes is generally associated with DNA methylation, gene silencing, and decreased gene expression (Davie & Chadee, 1998). The relationship between patterns of histone modifications and gene activity provides evidence for the existence of a “histone code” for determining cell-specific gene expression programs (Jenuwein & Allis, 2001). Interestingly, recent research using animal models has demonstrated that histone modifications and DNA methylation of certain genes mediates the long-term behavioral effects of the level of care experienced during infancy.
Early childhood experience
The development of an individual is an active process of adaptation that occurs within a social and economic context. For example, the closeness or degree of positive attachment of the parent (typically mother)–infant bond and parental investment (including nutrient supply provided by the parent) that define early childhood experience also program the development of individual differences in stress responses in the brain, which then affect memory, attention, and emotion. In terms of evolution, this process provides the offspring with the ability to physiologically adjust gene expression profiles contributing to the organization and function of neural circuits and molecular pathways that support (1) biological defensive systems for survival (e.g., stress resilience), (2) reproductive success to promote establishment and persistence in the present environment, and (3) adequate parenting in the next generation (Bradshaw, 1965).
Parental investment and programming of stress responses in the offspring
The most comprehensive study to date of variations in parental investment and epigenetic inheritance in mammals is that of the maternally transmitted responses to stress in rats. In rat pups, maternal nurturing (licking and grooming) during the first week of life is associated with long-term programming of individual differences in stress responsiveness, emotionality, cognitive performance, and reproductive behavior (Caldji et al., 1998; Francis, Diorio, Liu, & Meaney, 1999; Liu et al., 1997; Myers, Brunelli, Shair, Squire, & Hofer, 1989; Stern, 1997). In adulthood, the offspring of mothers that exhibit increased levels of pup licking and grooming over the first week of life show increased expression of the glucocorticoid receptor in the hippocampus (a brain structure associated with stress responsivity as well as learning and memory) and a lower hormonal response to stress compared with adult animals reared by low licking and grooming mothers (Francis et al., 1999; Liu et al., 1997). Moreover, rat pups that received low levels of maternal licking and grooming during the first week of life showed decreased histone acetylation and increased DNA methylation of a neuron-specific promoter of the glucocorticoid receptor gene (Weaver et al., 2004). The expression of this gene is then reduced, the number of glucocorticoid receptors in the brain is decreased, and the animals show a higher hormonal response to stress throughout their life. The effects of maternal care on stress hormone responses and behaviour in the offspring can be eliminated in adulthood by pharmacological treatment (HDAC inhibitor trichostatin A, TSA) or dietary amino acid supplementation (methyl donor L-methionine), treatments that influence histone acetylation, DNA methylation, and expression of the glucocorticoid receptor gene (Weaver et al., 2004; Weaver et al., 2005). This series of experiments shows that histone acetylation and DNA methylation of the glucocorticoid receptor gene promoter is a necessary link in the process leading to the long-term physiological and behavioral sequelae of poor maternal care. This points to a possible molecular target for treatments that may reverse or ameliorate the traces of childhood maltreatment.
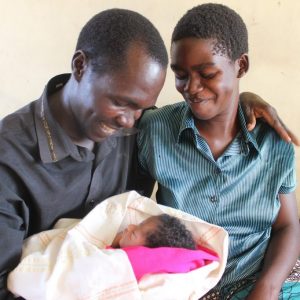
Several studies have attempted to determine to what extent the findings from model animals are transferable to humans. Examination of post-mortem brain tissue from healthy human subjects found that the human equivalent of the glucocorticoid receptor gene promoter (NR3C1 exon 1F promoter) is also unique to the individual (Turner, Pelascini, Macedo, & Muller, 2008). A similar study examining newborns showed that methylation of the glucocorticoid receptor gene promoter maybe an early epigenetic marker of maternal mood and risk of increased hormonal responses to stress in infants 3 months of age (Oberlander et al., 2008). Although further studies are required to examine the functional consequence of this DNA methylation, these findings are consistent with our studies in the neonate and adult offspring of low licking and grooming mothers that show increased DNA methylation of the promoter of the glucocorticoid receptor gene, decreased glucocorticoid receptor gene expression, and increased hormonal responses to stress (Weaver et al., 2004). Examination of brain tissue from suicide victims found that the human glucocorticoid receptor gene promoter is also more methylated in the brains of individuals who had experienced maltreatment during childhood (McGowan et al., 2009). These finding suggests that DNA methylation mediates the effects of early environment in both rodents and humans and points to the possibility of new therapeutic approaches stemming from translational epigenetic research. Indeed, similar processes at comparable epigenetic labile regions could explain why the adult offspring of high and low licking/grooming mothers exhibit widespread differences in hippocampal gene expression and cognitive function (Weaver, Meaney, & Szyf, 2006).
However, this type of research is limited by the inaccessibility of human brain samples. The translational potential of this finding would be greatly enhanced if the relevant epigenetic modification can be measured in an accessible tissue. Examination of blood samples from adult patients with bipolar disorder, who also retrospectively reported on their experiences of childhood abuse and neglect, found that the degree of DNA methylation of the human glucocorticoid receptor gene promoter was strongly positively related to the reported experience of childhood maltreatment decades earlier. For a relationship between a molecular measure and reported historical exposure, the effects size is extraordinarily large. This opens a range of new possibilities: given the large effect size and consistency of this association, measurement of the GR promoter methylation may effectively become a blood test measuring the physiological traces left on the genome by early experiences. Although this blood test cannot replace current methods of diagnosis, this unique and addition information adds to our knowledge of how disease may arise and be manifested throughout life. Near-future research will examine whether this measure adds value over and above simple reporting of early adversities when it comes to predicting important outcomes, such as response to treatment or suicide.
Child nutrition and the epigenome
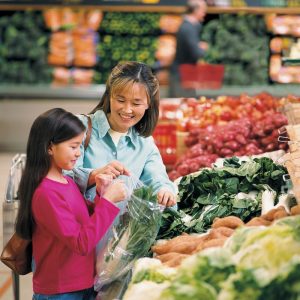
The old adage “you are what you eat” might be true on more than just a physical level: The food you choose (and even what your parents and grandparents chose) is reflected in your own personal development and risk for disease in adult life (Wells, 2003). Nutrients can reverse or change DNA methylation and histone modifications, thereby modifying the expression of critical genes associated with physiologic and pathologic processes, including embryonic development, aging, and carcinogenesis. It appears that nutrients can influence the epigenome either by directly inhibiting enzymes that catalyze DNA methylation or histone modifications, or by altering the availability of substrates necessary for those enzymatic reactions. For example, rat mothers fed a diet low in methyl group donors during pregnancy produce offspring with reduced DNMT-1 expression, decreased DNA methylation, and increased histone acetylation at promoter regions of specific genes, including the glucocorticoid receptor, and increased gene expression in the liver of juvenile offspring (Lillycrop, Phillips, Jackson, Hanson, & Burdge, 2005) and adult offspring (Lillycrop et al., 2007). These data suggest that early life nutrition has the potential to influence epigenetic programming in the brain not only during early development but also in adult life, thereby modulating health throughout life. In this regard, nutritional epigenetics has been viewed as an attractive tool to prevent pediatric developmental diseases and cancer, as well as to delay aging-associated processes.
The best evidence relating to the impact of adverse environmental conditions development and health comes from studies of the children of women who were pregnant during two civilian famines of World War II: the Siege of Leningrad (1941–44) (Bateson, 2001) and the Dutch Hunger Winter (1944–1945) (Stanner et al., 1997). In the Netherlands famine, women who were previously well nourished were subjected to low caloric intake and associated environmental stressors. Women who endured the famine in the late stages of pregnancy gave birth to smaller babies (Lumey & Stein, 1997) and these children had an increased risk of insulin resistance later in life (Painter, Roseboom, & Bleker, 2005). In addition, offspring who were starved prenatally later experienced impaired glucose tolerance in adulthood, even when food was more abundant (Stanner et al., 1997). Famine exposure at various stages of gestation was associated with a wide range of risks such as increased obesity, higher rates of coronary heart disease, and lower birth weight (Lumey & Stein, 1997). Interestingly, when examined 60 years later, people exposed to famine prenatally showed reduced DNA methylation compared with their unexposed same-sex siblings (Heijmans et al., 2008).
Epigenetic regulation of learning and memory
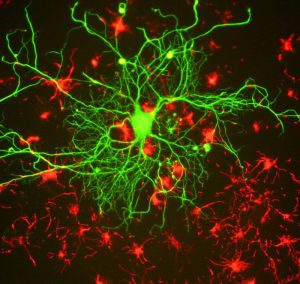
Memories are recollections of actual events stored within our brains. But how is our brain able to form and store these memories? Epigenetic mechanisms influence genomic activities in the brain to produce long-term changes in synaptic signaling, organization, and morphology, which in turn support learning and memory (Day & Sweatt, 2011).
Neuronal activity in the hippocampus of mice is associated with changes in DNA methylation (Guo et al., 2011), and disruption to genes encoding the DNA methylation machinery cause learning and memory impairments (Feng et al., 2010). DNA methylation has also been implicated in the maintenance of long-term memories, as pharmacological inhibition of DNA methylation and impaired memory (Day & Sweatt, 2011; Miller et al., 2010). These findings indicate the importance of DNA methylation in mediating synaptic plasticity and cognitive functions, both of which are disturbed in psychological illness.
Changes in histone modifications can also influence long-term memory formation by altering chromatin accessibility and the expression of genes relevant to learning and memory. Memory formation and the associated enhancements in synaptic transmission are accompanied by increases in histone acetylation (Guan et al., 2002) and alterations in histone methylation (Schaefer et al., 2009), which promote gene expression. Conversely, a neuronal increase in histone deacetylase activity, which promotes gene silencing, results in reduced synaptic plasticity and impairs memory (Guan et al., 2009). Pharmacological inhibition of histone deacetylases augments memory formation (Guan et al., 2009; Levenson et al., 2004), further suggesting that histone (de)acetylation regulates this process.
In humans genetic defects in genes encoding the DNA methylation and chromatin machinery exhibit profound effects on cognitive function and mental health (Jiang, Bressler, & Beaudet, 2004). The two best-characterized examples are Rett syndrome (Amir et al., 1999) and Rubinstein-Taybi syndrome (RTS) (Alarcon et al., 2004), which are profound intellectual disability disorders. Both MECP2 and CBP are highly expressed in neurons and are involved in regulating neural gene expression (Chen et al., 2003; Martinowich et al., 2003).
Rett syndrome patients have a mutation in their DNA sequence in a gene called MECP2. MECP2 plays many important roles within the cell: One of these roles is to read the DNA sequence, checking for DNA methylation, and to bind to areas that contain methylation, thereby preventing the wrong proteins from being present. Other roles for MECP2 include promoting the presence of particular, necessary, proteins, ensuring that DNA is packaged properly within the cell and assisting with the production of proteins. MECP2 function also influences gene expression that supports dendritic and synaptic development and hippocampus-dependent memory (Li, Zhong, Chau, Williams, & Chang, 2011; Skene et al., 2010). Mice with altered MECP2 expression exhibit genome-wide increases in histone acetylation, neuron cell death, increased anxiety, cognitive deficits, and social withdrawal (Shahbazian et al., 2002). These findings support a model in which DNA methylation and MECP2 constitute a cell-specific epigenetic mechanism for regulation of histone modification and gene expression, which may be disrupted in Rett syndrome.
RTS patients have a mutation in their DNA sequence in a gene called CBP. One of these roles of CBP is to bind to specific histones and promote histone acetylation, thereby promoting gene expression. Consistent with this function, RTS patients exhibit a genome-wide decrease in histone acetylation and cognitive dysfunction in adulthood (Kalkhoven et al., 2003). The learning and memory deficits are attributed to disrupted neural plasticity (Korzus, Rosenfeld, & Mayford, 2004). Similar to RTS in humans, mice with a mutation of CBP perform poorly in cognitive tasks and show decreased genome-wide histone acetylation (for review, see Josselyn, 2005). In the mouse brain CBP was found to act as an epigenetic switch to promote the birth of new neurons in the brain. Interestingly, this epigenetic mechanism is disrupted in the fetal brains of mice with a mutation of CBP, which, as pups, exhibit early behavioral deficits following removal and separation from their mother (Wang et al., 2010). These findings provide a novel mechanism whereby environmental cues, acting through histone modifying enzymes, can regulate epigenetic status and thereby directly promote neurogenesis, which regulates neurobehavioral development.
Together, these studies demonstrate that misregulation of epigenetic modifications and their regulatory enzymes is capable of orchestrating prominent deficits in neuronal plasticity and cognitive function. Knowledge from these studies may provide greater insight into other mental disorders such as depression and suicidal behaviors.
Epigenetic mechanisms in psychological disorders
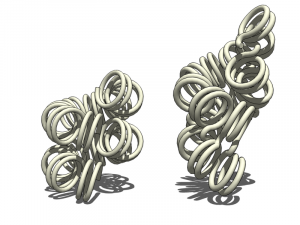
Epigenome-wide studies have identified several dozen sites with DNA methylation alterations in genes involved in brain development and neurotransmitter pathways, which had previously been associated with mental illness (Mill et al., 2008). These disorders are complex and typically start at a young age and cause lifelong disability. Often, limited benefits from treatment make these diseases some of the most burdensome disorders for individuals, families, and society. It has become evident that the efforts to identify the primary causes of complex psychiatric disorders may significantly benefit from studies linking environmental effects with changes observed within the individual cells.
Epigenetic events that alter chromatin structure to regulate programs of gene expression have been associated with depression-related behavior and action of antidepressant medications, with increasing evidence for similar mechanisms occurring in post-mortem brains of depressed individuals. In mice, social avoidance resulted in decreased expression of hippocampal genes important in mediating depressive responses (Tsankova et al., 2006). Similarly, chronic social defeat stress was found to decrease expression of genes implicated in normal emotion processing (Lutter et al., 2008). Consistent with these findings, levels of histone markers of increased gene expression were down regulated in human post-mortem brain samples from individuals with a history of clinical depression (Covington et al., 2009).
Administration of antidepressants increased histone markers of increased gene expression and reversed the gene repression induced by defeat stress (Lee, Wynder, Schmidt, McCafferty, & Shiekhattar, 2006; Tsankova et al., 2006; Wilkinson et al., 2009). These results provide support for the use of HDAC inhibitors against depression. Accordingly, several HDAC inhibitors have been found to exert antidepressant effects by each modifying distinct cellular targets (Cassel et al., 2006; Schroeder, Lin, Crusio, & Akbarian, 2007).
There is also increasing evidence that aberrant gene expression resulting from altered epigenetic regulation is associated with the pathophysiology of suicide (McGowan et al., 2008; Poulter et al., 2008). Thus, it is tempting to speculate that there is an epigenetically determined reduced capacity for gene expression, which is required for learning and memory, in the brains of suicide victims.
Epigenetic strategy to understanding gene-environment interactions
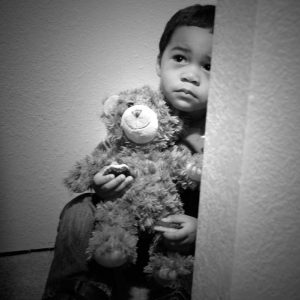
While the cellular and molecular mechanisms that influence on physical and mental health have long been a central focus of neuroscience, only in recent years has attention turned to the epigenetic mechanisms behind the dynamic changes in gene expression responsible for normal cognitive function and increased risk for mental illness. The links between early environment and epigenetic modifications suggest a mechanism underlying gene-environment interactions. Early environmental adversity alone is not a sufficient cause of mental illness, because many individuals with a history of severe childhood maltreatment or trauma remain healthy. It is increasingly becoming evident that inherited differences in the segments of specific genes may moderate the effects of adversity and determine who is sensitive and who is resilient through a gene-environment interplay. Genes such as the glucocorticoid receptor appear to moderate the effects of childhood adversity on mental illness. Remarkably, epigenetic DNA modifications have been identified that may underlie the long-lasting effects of environment on biological functions. This new epigenetic research is pointing to a new strategy to understanding gene-environment interactions.
The next decade of research will show if this potential can be exploited in the development of new therapeutic options that may alter the traces that early environment leaves on the genome. However, as discussed in this module, the epigenome is not static and can be molded by developmental signals, environmental perturbations, and disease states, which present an experimental challenge in the search for epigenetic risk factors in psychological disorders (Rakyan, Down, Balding, & Beck, 2011). The sample size and epigenomic assay required is dependent on the number of tissues affected, as well as the type and distribution of epigenetic modifications. The combination of genetic association maps studies with epigenome-wide developmental studies may help identify novel molecular mechanisms to explain features of inheritance of personality traits and transform our understanding of the biological basis of psychology. Importantly, these epigenetic studies may lead to identification of novel therapeutic targets and enable the development of improved strategies for early diagnosis, prevention, and better treatment of psychological and behavioral disorders.
A “Big Picture” Insight into Epi-Genetics
Epigentics is a content area where students sometimes feel apprehensive, especially if they do not have a background in biology. This course will teach you all required biology content expected for this class. To help get you excited about the importance of this research field, please watch the following video. It provides insights into why it is so important for behavioural scientists to understand, at least on a surface level, about the field of epigenetics. After you watch it, please be sure to go through this module again. This video is not intended as a replacement for the content in this module, but rather is intended to serve as a big-picture framework for you to better understand the content in this module.
Check Your Knowledge
To help you with your studying, we’ve included some practice questions for this module. These questions do not necessarily address all content in this module. They are intended as practice, and you are responsible for all of the content in this module even if there is no associated practice question. To promote deeper engagement with the material, we encourage you to create some questions of your own for your practice. You can then also return to these self-generated questions later in the course to test yourself.
Vocabulary
- DNA methylation
- Covalent modifications of mammalian DNA occurring via the methylation of cytosine, typically in the context of the CpG dinucleotide.
- DNA methyltransferases (DNMTs)
- Enzymes that establish and maintain DNA methylation using methyl-group donor compounds or cofactors. The main mammalian DNMTs are DNMT1, which maintains methylation state across DNA replication, and DNMT3a and DNMT3b, which perform de novo methylation.
- Epigenetics
- The study of heritable changes in gene expression or cellular phenotype caused by mechanisms other than changes in the underlying DNA sequence. Epigenetic marks include covalent DNA modifications and posttranslational histone modifications.
- Epigenome
- The genome-wide distribution of epigenetic marks.
- Gene
- A specific deoxyribonucleic acid (DNA) sequence that codes for a specific polypeptide or protein or an observable inherited trait.
- Genome-wide association study (GWAS)
- A study that maps DNA polymorphisms in affected individuals and controls matched for age, sex, and ethnic background with the aim of identifying causal genetic variants.
- Genotype
- The DNA content of a cell’s nucleus, whether a trait is externally observable or not.
- Histone acetyltransferases (HATs) and histone deacetylases (HDACs)
- HATs are enzymes that transfer acetyl groups to specific positions on histone tails, promoting an “open” chromatin state and transcriptional activation. HDACs remove these acetyl groups, resulting in a “closed” chromatin state and transcriptional repression.
- Histone modifications
- Posttranslational modifications of the N-terminal “tails” of histone proteins that serve as a major mode of epigenetic regulation. These modifications include acetylation, phosphorylation, methylation, sumoylation, ubiquitination, and ADP-ribosylation.
- Identical twins
- Two individual organisms that originated from the same zygote and therefore are genetically identical or very similar. The epigenetic profiling of identical twins discordant for disease is a unique experimental design as it eliminates the DNA sequence-, age-, and sex-differences from consideration.
- Phenotype
- The pattern of expression of the genotype or the magnitude or extent to which it is observably expressed—an observable characteristic or trait of an organism, such as its morphology, development, biochemical or physiological properties, or behavior.
References
- Adams, R. L., McKay, E. L., Craig, L. M., & Burdon, R. H. (1979). Mouse DNA methylase: methylation of native DNA. Biochimica et Biophysica Acta, 561(2), 345–357.
- Alarcon, J. M., Malleret, G., Touzani, K., Vronskaya, S., Ishii, S., Kandel, E. R., & Barco, A. (2004). Chromatin acetylation, memory, and LTP are impaired in CBP+/- mice: a model for the cognitive deficit in Rubinstein-Taybi syndrome and its amelioration. Neuron, 42(6), 947–959. doi: 10.1016/j.neuron.2004.05.021, S0896627304003022 [pii]
- Amir, R. E., Van den Veyver, I. B., Wan, M., Tran, C. Q., Francke, U., & Zoghbi, H. Y. (1999). Rett syndrome is caused by mutations in X-linked MECP2, encoding methyl-CpG-binding protein 2. Nature Genetics, 23(2), 185–188.
- Bateson, P. (2001). Fetal experience and good adult design. International Journal of Epidemiology, 30(5), 928–934.
- Bradshaw, A.D. (1965). Evolutionary significance of phenotypic plasticity in plants. Advances in Genetics, 13, 115–155.
- Caldji, C., Tannenbaum, B., Sharma, S., Francis, D., Plotsky, P. M., & Meaney, M. J. (1998). Maternal care during infancy regulates the development of neural systems mediating the expression of fearfulness in the rat. Proceedings of the National Academy of Sciences U S A, 95(9), 5335–5340.
- Cassel, S., Carouge, D., Gensburger, C., Anglard, P., Burgun, C., Dietrich, J. B., . . . Zwiller, J. (2006). Fluoxetine and cocaine induce the epigenetic factors MeCP2 and MBD1 in adult rat brain. Molecular Pharmacology, 70(2), 487–492. doi: 10.1124/mol.106.022301
- Chen, W. G., Chang, Q., Lin, Y., Meissner, A., West, A. E., Griffith, E. C., . . . Greenberg, M. E. (2003). Derepression of BDNF transcription involves calcium-dependent phosphorylation of MeCP2. Science, 302(5646), 885–889.
- Covington, H. E., 3rd, Maze, I., LaPlant, Q. C., Vialou, V. F., Ohnishi, Y. N., Berton, O., . . . Nestler, E. J. (2009). Antidepressant actions of histone deacetylase inhibitors. Journal of Neuroscience, 29(37), 11451–11460. doi: 10.1523/JNEUROSCI.1758-09.2009
- Davie, J. R., & Chadee, D. N. (1998). Regulation and regulatory parameters of histone modifications. *Journal of Cellular Biochemistry Suppl, 30–31 *, 203–213.
- Day, J. J., & Sweatt, J. D. (2011). Epigenetic mechanisms in cognition. Neuron, 70(5), 813–829. doi: 10.1016/j.neuron.2011.05.019
- Dick, D. M., Riley, B., & Kendler, K. S. (2010). Nature and nurture in neuropsychiatric genetics: where do we stand? Dialogues in Clinical Neuroscience, 12(1), 7–23.
- Dolinoy, D. C., Weidman, J. R., & Jirtle, R. L. (2007). Epigenetic gene regulation: linking early developmental environment to adult disease. Reproductive Toxicology, 23(3), 297–307. doi: S0890-6238(06)00197-3 [pii], 10.1016/j.reprotox.2006.08.012
- Feng, J., Chang, H., Li, E., & Fan, G. (2005). Dynamic expression of de novo DNA methyltransferases Dnmt3a and Dnmt3b in the central nervous system. Journal of Neuroscience Research, 79(6), 734–746. doi: 10.1002/jnr.20404
- Feng, J., Zhou, Y., Campbell, S. L., Le, T., Li, E., Sweatt, J. D., . . . Fan, G. (2010). Dnmt1 and Dnmt3a maintain DNA methylation and regulate synaptic function in adult forebrain neurons. Nature Neuroscience, 13(4), 423–430. doi: 10.1038/nn.2514
- Francis, D., Diorio, J., Liu, D., & Meaney, M. J. (1999). Nongenomic transmission across generations of maternal behavior and stress responses in the rat. Science, 286(5442), 1155–1158.
- Gershon, E. S., Alliey-Rodriguez, N., & Liu, C. (2011). After GWAS: searching for genetic risk for schizophrenia and bipolar disorder. American Journal of Psychiatry, 168(3), 253–256. doi: 10.1176/appi.ajp.2010.10091340
- Goll, M. G., & Bestor, T. H. (2005). Eukaryotic cytosine methyltransferases. Annual Review of Biochemistry, 74, 481–514. doi: 10.1146/annurev.biochem.74.010904.153721
- Goto, K., Numata, M., Komura, J. I., Ono, T., Bestor, T. H., & Kondo, H. (1994). Expression of DNA methyltransferase gene in mature and immature neurons as well as proliferating cells in mice. Differentiation, 56(1–2), 39–44.
- Gregg, C., Zhang, J., Weissbourd, B., Luo, S., Schroth, G. P., Haig, D., & Dulac, C. (2010). High-resolution analysis of parent-of-origin allelic expression in the mouse brain. Science, 329(5992), 643–648. doi: 10.1126/science.1190830
- Guan, J. S., Haggarty, S. J., Giacometti, E., Dannenberg, J. H., Joseph, N., Gao, J., . . . Tsai, L. H. (2009). HDAC2 negatively regulates memory formation and synaptic plasticity. Nature, 459(7243), 55-60. doi: 10.1038/nature07925
- Guan, Z., Giustetto, M., Lomvardas, S., Kim, J. H., Miniaci, M. C., Schwartz, J. H., . . . Kandel, E. R. (2002). Integration of long-term-memory-related synaptic plasticity involves bidirectional regulation of gene expression and chromatin structure. Cell, 111(4), 483–493.
- Guo, J. U., Ma, D. K., Mo, H., Ball, M. P., Jang, M. H., Bonaguidi, M. A., . . . Song, H. (2011). Neuronal activity modifies the DNA methylation landscape in the adult brain. Nature Neuroscience, 14(10), 1345–1351. doi: 10.1038/nn.2900
- Heijmans, B. T., Tobi, E. W., Stein, A. D., Putter, H., Blauw, G. J., Susser, E. S., . . . Lumey, L. H. (2008). Persistent epigenetic differences associated with prenatal exposure to famine in humans. Proceedings of the National Academy of Sciences U S A, 105(44), 17046–17049. doi: 0806560105 [pii]10.1073/pnas.0806560105
- Hong, L., Schroth, G. P., Matthews, H. R., Yau, P., & Bradbury, E. M. (1993). Studies of the DNA binding properties of histone H4 amino terminus. Thermal denaturation studies reveal that acetylation markedly reduces the binding constant of the H4 “tail” to DNA. Journal of Biological Chemistry, 268(1), 305–314.
- Jenuwein, T., & Allis, C. D. (2001). Translating the histone code. Science, 293(5532), 1074–1080. doi: 10.1126/Science.1063127293/5532/1074 [pii]
- Jiang, Y. H., Bressler, J., & Beaudet, A. L. (2004). Epigenetics and human disease. Annual Review of Genomics and Human Genetics, 5, 479–510. doi: 10.1146/annurev.genom.5.061903.180014
- Josselyn, S. A. (2005). What’s right with my mouse model? New insights into the molecular and cellular basis of cognition from mouse models of Rubinstein-Taybi Syndrome. Learning & Memory, 12(2), 80–83. doi: 12/2/80 [pii]10.1101/lm.93505
- Kadonaga, J. T. (1998). Eukaryotic transcription: an interlaced network of transcription factors and chromatin-modifying machines. Cell, 92(3), 307–313.
- Kalkhoven, E., Roelfsema, J. H., Teunissen, H., den Boer, A., Ariyurek, Y., Zantema, A., . . . Peters, D. J. (2003). Loss of CBP acetyltransferase activity by PHD finger mutations in Rubinstein-Taybi syndrome. Human Molecular Genetics, 12(4), 441–450.
- Korzus, E., Rosenfeld, M. G., & Mayford, M. (2004). CBP histone acetyltransferase activity is a critical component of memory consolidation. Neuron, 42(6), 961–972. doi: 10.1016/j.neuron.2004.06.002S0896627304003526 [pii]
- Kuo, M. H., & Allis, C. D. (1998). Roles of histone acetyltransferases and deacetylases in gene regulation. Bioessays, 20(8), 615–626. doi: 10.1002/(SICI)1521–1878(199808)20:8<615::AID-BIES4>3.0.CO;2-H [pii] 10.1002/(SICI)1521-1878(199808)20:8<615::AID-BIES4>3.0.CO;2-H
- Law, J. A., & Jacobsen, S. E. (2010). Establishing, maintaining and modifying DNA methylation patterns in plants and animals. Nature Reviews Genetics, 11(3), 204–220. doi: nrg2719 [pii]10.1038/nrg2719
- Lee, M. G., Wynder, C., Schmidt, D. M., McCafferty, D. G., & Shiekhattar, R. (2006). Histone H3 lysine 4 demethylation is a target of nonselective antidepressive medications. Chemistry & Biology, 13(6), 563–567. doi: 10.1016/j.chembiol.2006.05.004
- Levenson, J. M., O’Riordan, K. J., Brown, K. D., Trinh, M. A., Molfese, D. L., & Sweatt, J. D. (2004). Regulation of histone acetylation during memory formation in the hippocampus. Journal of Biological Chemistry, 279(39), 40545–40559.
- Li, H., Zhong, X., Chau, K. F., Williams, E. C., & Chang, Q. (2011). Loss of activity-induced phosphorylation of MeCP2 enhances synaptogenesis, LTP and spatial memory. Nature Neuroscience, 14(8), 1001–1008. doi: 10.1038/nn.2866
- Lillycrop, K. A., Phillips, E. S., Jackson, A. A., Hanson, M. A., & Burdge, G. C. (2005). Dietary protein restriction of pregnant rats induces and folic acid supplementation prevents epigenetic modification of hepatic gene expression in the offspring. Journal of Nutrition, 135(6), 1382–1386. doi: 135/6/1382 [pii]
- Lillycrop, K. A., Slater-Jefferies, J. L., Hanson, M. A., Godfrey, K. M., Jackson, A. A., & Burdge, G. C. (2007). Induction of altered epigenetic regulation of the hepatic glucocorticoid receptor in the offspring of rats fed a protein-restricted diet during pregnancy suggests that reduced DNA methyltransferase-1 expression is involved in impaired DNA methylation and changes in histone modifications. British Journal of Nutrition, 97(6), 1064–1073. doi: S000711450769196X [pii]10.1017/S000711450769196X
- Liu, D., Diorio, J., Tannenbaum, B., Caldji, C., Francis, D., Freedman, A., . . . Meaney, M. J. (1997). Maternal care, hippocampal glucocorticoid receptors, and hypothalamic- pituitary-adrenal responses to stress [see comments]. Science, 277(5332), 1659–1662.
- Lumey, L. H., & Stein, A. D. (1997). Offspring birth weights after maternal intrauterine undernutrition: a comparison within sibships. American Journal of Epidemiology, 146(10), 810–819.
- Lutter, M., Krishnan, V., Russo, S. J., Jung, S., McClung, C. A., & Nestler, E. J. (2008). Orexin signaling mediates the antidepressant-like effect of calorie restriction. Journal of Neuroscience, 28(12), 3071–3075. doi: 10.1523/JNEUROSCI.5584-07.2008
- Martinowich, K., Hattori, D., Wu, H., Fouse, S., He, F., Hu, Y., . . . Sun, Y. E. (2003). DNA methylation-related chromatin remodeling in activity-dependent BDNF gene regulation. Science, 302(5646), 890–893.
- McGowan, P. O., Sasaki, A., D’Alessio, A. C., Dymov, S., Labonte, B., Szyf, M., . . . Meaney, M. J. (2009). Epigenetic regulation of the glucocorticoid receptor in human brain associates with childhood abuse. Nature Neuroscience, 12(3), 342–348. doi: nn.2270 [pii]10.1038/nn.2270
- McGowan, P. O., Sasaki, A., Huang, T. C., Unterberger, A., Suderman, M., Ernst, C., . . . Szyf, M. (2008). Promoter-wide hypermethylation of the ribosomal RNA gene promoter in the suicide brain. PLoS ONE, 3(5), e2085. doi: 10.1371/journal.pone.0002085
- Mill, J., Tang, T., Kaminsky, Z., Khare, T., Yazdanpanah, S., Bouchard, L., . . . Petronis, A. (2008). Epigenomic profiling reveals DNA-methylation changes associated with major psychosis. American Journal of Human Genetics, 82(3), 696–711. doi: 10.1016/j.ajhg.2008.01.008
- Miller, C. A., Gavin, C. F., White, J. A., Parrish, R. R., Honasoge, A., Yancey, C. R., . . . Sweatt, J. D. (2010). Cortical DNA methylation maintains remote memory. Nature Neuroscience, 13(6), 664–666. doi: 10.1038/nn.2560
- Myers, M. M., Brunelli, S. A., Shair, H. N., Squire, J. M., & Hofer, M. A. (1989). Relationships between maternal behavior of SHR and WKY dams and adult blood pressures of cross-fostered F1 pups. Developmental Psychobiology, 22(1), 55–67.
- Oberlander, T. F., Weinberg, J., Papsdorf, M., Grunau, R., Misri, S., & Devlin, A. M. (2008). Prenatal exposure to maternal depression, neonatal methylation of human glucocorticoid receptor gene (NR3C1) and infant cortisol stress responses. Epigenetics, 3(2), 97–106. doi: 6034 [pii]
- Ooi, S. K., O’Donnell, A. H., & Bestor, T. H. (2009). Mammalian cytosine methylation at a glance. Journal of Cell Science, 122(Pt 16), 2787–2791. doi: 122/16/2787 [pii]10.1242/jcs.015123
- Painter, R. C., Roseboom, T. J., & Bleker, O. P. (2005). Prenatal exposure to the Dutch famine and disease in later life: an overview. Reproductive Toxicology, 20(3), 345–352. doi: S0890-6238(05)00088-2 [pii]10.1016/j. Reproductive Toxicology.2005.04.005
- Petronis, A. (2010). Epigenetics as a unifying principle in the aetiology of complex traits and diseases. Nature, 465(7299), 721–727. doi: 10.1038/nature09230
- Poulter, M. O., Du, L., Weaver, I. C., Palkovits, M., Faludi, G., Merali, Z., . . . Anisman, H. (2008). GABAA receptor promoter hypermethylation in suicide brain: implications for the involvement of epigenetic processes. Biological Psychiatry, 64(8), 645–652. doi: 10.1016/j.biopsych.2008.05.028
- Rakyan, V. K., Down, T. A., Balding, D. J., & Beck, S. (2011). Epigenome-wide association studies for common human diseases. Nature Reviews Genetics, 12(8), 529–541. doi: 10.1038/nrg3000
- Razin, A. (1998). CpG methylation, chromatin structure and gene silencing-a three-way connection. European Molecular Biology Organization, 17(17), 4905–4908.
- Schaefer, A., Sampath, S. C., Intrator, A., Min, A., Gertler, T. S., Surmeier, D. J., . . . Greengard, P. (2009). Control of cognition and adaptive behavior by the GLP/G9a epigenetic suppressor complex. Neuron, 64(5), 678–691. doi: 10.1016/j.neuron.2009.11.019
- Schroeder, F. A., Lin, C. L., Crusio, W. E., & Akbarian, S. (2007). Antidepressant-like effects of the histone deacetylase inhibitor, sodium butyrate, in the mouse. Biological Psychiatry, 62(1), 55-64. doi: 10.1016/j.biopsych.2006.06.036
- Sealy, L., & Chalkley, R. (1978). DNA associated with hyperacetylated histone is preferentially digested by DNase I. Nucleic Acids Research, 5(6), 1863–1876.
- Shahbazian, M., Young, J., Yuva-Paylor, L., Spencer, C., Antalffy, B., Noebels, J., . . . Zoghbi, H. (2002). Mice with truncated MeCP2 recapitulate many Rett syndrome features and display hyperacetylation of histone H3. Neuron, 35(2), 243–254.
- Skene, P. J., Illingworth, R. S., Webb, S., Kerr, A. R., James, K. D., Turner, D. J., . . . Bird, A. P. (2010). Neuronal MeCP2 is expressed at near histone-octamer levels and globally alters the chromatin state. Molecular Cell, 37(4), 457–468. doi: 10.1016/j.molcel.2010.01.030
- Stanner, S. A., Bulmer, K., Andres, C., Lantseva, O. E., Borodina, V., Poteen, V. V., & Yudkin, J. S. (1997). Does malnutrition in utero determine diabetes and coronary heart disease in adulthood? Results from the Leningrad siege study, a cross sectional study. British Medical Journal, 315(7119), 1342–1348.
- Stern, J. M. (1997). Offspring-induced nurturance: animal-human parallels. Developmental Psychobiololgy, 31(1), 19–37.
- Sutter, D., Doerfler, W., 1980. Methylation of integrated adenovirus type 12 DNA sequences in transformed cells is inversely correlated with viral gene expression. Proceedings of the National Academy of Sciences U S A. 77, 253–256.
- Suzuki, M. M., & Bird, A. (2008). DNA methylation landscapes: provocative insights from epigenomics. Nature Reviews Genetics, 9(6), 465–476. doi: nrg2341 [pii]10.1038/nrg2341
- Tsankova, N. M., Berton, O., Renthal, W., Kumar, A., Neve, R. L., & Nestler, E. J. (2006). Sustained hippocampal chromatin regulation in a mouse model of depression and antidepressant action. Nature Neuroscience. 9(4): 519–525. doi:10.1038/nn1659
- Turner, J. D., Pelascini, L. P., Macedo, J. A., & Muller, C. P. (2008). Highly individual methylation patterns of alternative glucocorticoid receptor promoters suggest individualized epigenetic regulatory mechanisms. Nucleic Acids Research, 36(22), 7207–7218. doi: gkn897 [pii] 10.1093/nar/gkn897
- Vardimon, L., Kressmann, A., Cedar, H., Maechler, M., Doerfler, W., 1982. Expression of a cloned adenovirus gene is inhibited by in vitro methylation. Proceedings of the National Academy of Sciences U S A. 79, 1073–1077.
- Waddington, C. H. (1942). Epigenotype. Endeavour(1), 18–21.
- Wade, P. A., Pruss, D., & Wolffe, A. P. (1997). Histone acetylation: chromatin in action. Trends in Biochemical Sciences, 22(4), 128–132. doi: S0968000497010165 [pii]
- Wang, J., Weaver, I. C., Gauthier-Fisher, A., Wang, H., He, L., Yeomans, J., . . . Miller, F. D. (2010). CBP histone acetyltransferase activity regulates embryonic neural differentiation in the normal and Rubinstein-Taybi syndrome brain. Developmental Cell, 18(1), 114–125. doi: 10.1016/j.devcel.2009.10.023
- Weaver, I. C., Cervoni, N., Champagne, F. A., D’Alessio, A. C., Sharma, S., Seckl, J. R., . . . Meaney, M. J. (2004). Epigenetic programming by maternal behavior. Nature Neuroscience, 7(8), 847–854. doi: 10.1038/nn1276
- Weaver, I. C., Champagne, F. A., Brown, S. E., Dymov, S., Sharma, S., Meaney, M. J., & Szyf, M. (2005). Reversal of maternal programming of stress responses in adult offspring through methyl supplementation: altering epigenetic marking later in life. Journal of Neuroscience, 25(47), 11045–11054. doi: 10.1523/JNEUROSCI.3652-05.2005
- Weaver, I. C., Meaney, M. J., & Szyf, M. (2006). Maternal care effects on the hippocampal transcriptome and anxiety-mediated behaviors in the offspring that are reversible in adulthood. Proceedings of the National Academy of Sciences U S A, 103(9), 3480–3485. doi: 10.1073/pnas.0507526103
- Wells, J. C. (2003). The thrifty phenotype hypothesis: thrifty offspring or thrifty mother? Journal of Theoretical Biology, 221(1), 143–161.
- Wilkinson, M. B., Xiao, G., Kumar, A., LaPlant, Q., Renthal, W., Sikder, D., . . . Nestler, E. J. (2009). Imipramine treatment and resiliency exhibit similar chromatin regulation in the mouse nucleus accumbens in depression models. Journal of Neuroscience, 29(24), 7820–7832. doi: 10.1523/JNEUROSCI.0932-09.2009
- Wolffe, A. P., & Matzke, M. A. (1999). Epigenetics: regulation through repression. Science, 286(5439), 481–486.
How to cite this Chapter using APA Style:
Weaver, I. (2019). Epigenetics in psychology. Adapted for use by Queen’s University. Original chapter in R. Biswas-Diener & E. Diener (Eds), Noba textbook series: Psychology.Champaign, IL: DEF publishers. Retrieved from http://noba.to/37p5cb8v
Copyright and Acknowledgment:
This material is licensed under the Creative Commons Attribution-NonCommercial-ShareAlike 4.0 International License. To view a copy of this license, visit: http://creativecommons.org/licenses/by-nc-sa/4.0/deed.en_US.
This material is attributed to the Diener Education Fund (copyright © 2018) and can be accessed via this link: http://noba.to/37p5cb8v.
Additional information about the Diener Education Fund (DEF) can be accessed here.
Original chapter by Ian Weaver adapted by Queen's University Psychology Department
This Open Access chapter was originally written for the NOBA project. Information on the NOBA project can be found below.
Early life experiences exert a profound and long-lasting influence on physical and mental health throughout life. The efforts to identify the primary causes of this have significantly benefited from studies of the epigenome—a dynamic layer of information associated with DNA that differs between individuals and can be altered through various experiences and environments. The epigenome has been heralded as a key “missing piece” of the etiological puzzle for understanding how development of psychological disorders may be influenced by the surrounding environment, in concordance with the genome. Understanding the mechanisms involved in the initiation, maintenance, and heritability of epigenetic states is thus an important aspect of research in current biology, particularly in the study of learning and memory, emotion, and social behavior in humans. Moreover, epigenetics in psychology provides a framework for understanding how the expression of genes is influenced by experiences and the environment to produce individual differences in behavior, cognition, personality, and mental health. In this module, we survey recent developments revealing epigenetic aspects of mental health and review some of the challenges of epigenetic approaches in psychology to help explain how nurture shapes nature.
Learning Objectives
- Explain what the term epigenetics means and the molecular machinery involved.
- Name and discuss important neural and developmental pathways that are regulated by epigenetic factors, and provide examples of epigenetic effects on personality traits and cognitive behavior.
- Understand how misregulation of epigenetic mechanisms can lead to disease states, and be able to discuss examples.
- Recognize how epigenetic machinery can be targets for therapeutic agents, and discuss examples.
Introduction
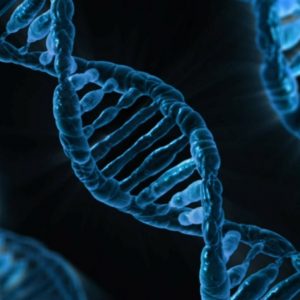
Early childhood is not only a period of physical growth; it is also a time of mental development related to changes in the anatomy, physiology, and chemistry of the nervous system that influence mental health throughout life. Cognitive abilities associated with learning and memory, reasoning, problem solving, and developing relationships continue to emerge during childhood. Brain development is more rapid during this critical or sensitive period than at any other, with more than 700 neural connections created each second. Herein, complex gene–environment interactions (or genotype–environment interactions, G×E) serve to increase the number of possible contacts between neurons, as they hone their adult synaptic properties and excitability. Many weak connections form to different neuronal targets; subsequently, they undergo remodeling in which most connections vanish and a few stable connections remain. These structural changes (or plasticity) may be crucial for the development of mature neural networks that support emotional, cognitive, and social behavior. The generation of different morphology, physiology, and behavioral outcomes from a single genome in response to changes in the environment forms the basis for “phenotypic plasticity,” which is fundamental to the way organisms cope with environmental variation, navigate the present world, and solve future problems.
The challenge for psychology has been to integrate findings from genetics and environmental (social, biological, chemical) factors, including the quality of infant–mother attachments, into the study of personality and our understanding of the emergence of mental illness. These studies have demonstrated that common DNA sequence variation and rare mutations account for only a small fraction (1%–2%) of the total risk for inheritance of personality traits and mental disorders (Dick, Riley, & Kendler, 2010; Gershon, Alliey-Rodriguez, & Liu, 2011). Additionally, studies that have attempted to examine the mechanisms and conditions under which DNA sequence variation influences brain development and function have been confounded by complex cause-and-effect relationships (Petronis, 2010). The large unaccounted heritability of personality traits and mental health suggests that additional molecular and cellular mechanisms are involved.
Epigenetics has the potential to provide answers to these important questions and refers to the transmission of phenotype in terms of gene expression in the absence of changes in DNA sequence—hence the name epi- (Greek: επί- over, above) genetics (Waddington, 1942; Wolffe & Matzke, 1999). The advent of high-throughput techniques such as sequencing-based approaches to study the distributions of regulators of gene expression throughout the genome led to the collective description of the “epigenome.” In contrast to the genome sequence, which is static and the same in almost all cells, the epigenome is highly dynamic, differing among cell types, tissues, and brain regions (Gregg et al., 2010). Recent studies have provided insights into epigenetic regulation of developmental pathways in response to a range of external environmental factors (Dolinoy, Weidman, & Jirtle, 2007). These environmental factors during early childhood and adolescence can cause changes in expression of genes conferring risk of mental health and chronic physical conditions. Thus, the examination of genetic–epigenetic–environment interactions from a developmental perspective may determine the nature of gene misregulation in psychological disorders.
This module will provide an overview of the main components of the epigenome and review themes in recent epigenetic research that have relevance for psychology, to form the biological basis for the interplay between environmental signals and the genome in the regulation of individual differences in physiology, emotion, cognition, and behavior.
Molecular control of gene expression: the dynamic epigenome
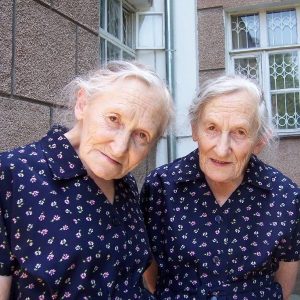
Almost all the cells in our body are genetically identical, yet our body generates many different cell types, organized into different tissues and organs, and expresses different proteins. Within each type of mammalian cell, about 2 meters of genomic DNA is divided into nuclear chromosomes. Yet the nucleus of a human cell, which contains the chromosomes, is only about 2 μm in diameter. To achieve this 1,000,000-fold compaction, DNA is wrapped around a group of 8 proteins called histones. This combination of DNA and histone proteins forms a special structure called a “nucleosome,” the basic unit of chromatin, which represents a structural solution for maintaining and accessing the tightly compacted genome. These factors alter the likelihood that a gene will be expressed or silenced. Cellular functions such as gene expression, DNA replication, and the generation of specific cell types are therefore influenced by distinct patterns of chromatin structure, involving covalent modification of both histones (Kadonaga, 1998) and DNA (Razin, 1998).
Importantly, epigenetic variation also emerges across the lifespan. For example, although identical twins share a common genotype and are genetically identical and epigenetically similar when they are young, as they age they become more dissimilar in their epigenetic patterns and often display behavioral, personality, or even physical differences, and have different risk levels for serious illness. Thus, understanding the structure of the nucleosome is key to understanding the precise and stable control of gene expression and regulation, providing a molecular interface between genes and environmentally induced changes in cellular activity.
The primary epigenetic mark: DNA modification
DNA methylation is the best-understood epigenetic modification influencing gene expression. DNA is composed of four types of naturally occurring nitrogenous bases: adenine (A), thymine (T), guanine (G), and cytosine (C). In mammalian genomes, DNA methylation occurs primarily at cytosine residues in the context of cytosines that are followed by guanines (CpG dinucleotides), to form 5-methylcytosine in a cell-specific pattern (Goll & Bestor, 2005; Law & Jacobsen, 2010; Suzuki & Bird, 2008). The enzymes that perform DNA methylation are called DNA methyltransferases (DNMTs), which catalyze the transfer of a methyl group to the cytosine (Adams, McKay, Craig, & Burdon, 1979). These enzymes are all expressed in the central nervous system and are dynamically regulated during development (Feng, Chang, Li, & Fan, 2005; Goto et al., 1994). The effect of DNA methylation on gene function varies depending on the period of development during which the methylation occurs and location of the methylated cytosine. Methylation of DNA in gene regulatory regions (promoter and enhancer regions) usually results in gene silencing and reduced gene expression (Ooi, O’Donnell, & Bestor, 2009; Suzuki & Bird, 2008; Sutter and Doerfler, 1980; Vardimon et al., 1982). This is a powerful regulatory mechanism that ensures that genes are expressed only when needed. Thus DNA methylation may broadly impact human brain development, and age-related misregulation of DNA methylation is associated with the molecular pathogenesis of neurodevelopmental disorders.
Histone modification and the histone code
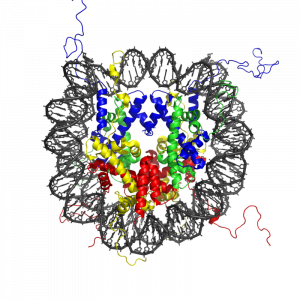
The modification of histone proteins comprises an important epigenetic mark related to gene expression. One of the most thoroughly studied modifications is histone acetylation, which is associated with gene activation and increased gene expression (Wade, Pruss, & Wolffe, 1997). Acetylation on histone tails is mediated by the opposing enzymatic activities of histone acetyltransferases (HATs) and histone deacetylases (HDACs) (Kuo & Allis, 1998). For example, acetylation of histone in gene regulatory regions by HAT enzymes is generally associated with DNA demethylation, gene activation, and increased gene expression (Hong, Schroth, Matthews, Yau, & Bradbury, 1993; Sealy & Chalkley, 1978). On the other hand, removal of the acetyl group (deacetylation) by HDAC enzymes is generally associated with DNA methylation, gene silencing, and decreased gene expression (Davie & Chadee, 1998). The relationship between patterns of histone modifications and gene activity provides evidence for the existence of a “histone code” for determining cell-specific gene expression programs (Jenuwein & Allis, 2001). Interestingly, recent research using animal models has demonstrated that histone modifications and DNA methylation of certain genes mediates the long-term behavioral effects of the level of care experienced during infancy.
Early childhood experience
The development of an individual is an active process of adaptation that occurs within a social and economic context. For example, the closeness or degree of positive attachment of the parent (typically mother)–infant bond and parental investment (including nutrient supply provided by the parent) that define early childhood experience also program the development of individual differences in stress responses in the brain, which then affect memory, attention, and emotion. In terms of evolution, this process provides the offspring with the ability to physiologically adjust gene expression profiles contributing to the organization and function of neural circuits and molecular pathways that support (1) biological defensive systems for survival (e.g., stress resilience), (2) reproductive success to promote establishment and persistence in the present environment, and (3) adequate parenting in the next generation (Bradshaw, 1965).
Parental investment and programming of stress responses in the offspring
The most comprehensive study to date of variations in parental investment and epigenetic inheritance in mammals is that of the maternally transmitted responses to stress in rats. In rat pups, maternal nurturing (licking and grooming) during the first week of life is associated with long-term programming of individual differences in stress responsiveness, emotionality, cognitive performance, and reproductive behavior (Caldji et al., 1998; Francis, Diorio, Liu, & Meaney, 1999; Liu et al., 1997; Myers, Brunelli, Shair, Squire, & Hofer, 1989; Stern, 1997). In adulthood, the offspring of mothers that exhibit increased levels of pup licking and grooming over the first week of life show increased expression of the glucocorticoid receptor in the hippocampus (a brain structure associated with stress responsivity as well as learning and memory) and a lower hormonal response to stress compared with adult animals reared by low licking and grooming mothers (Francis et al., 1999; Liu et al., 1997). Moreover, rat pups that received low levels of maternal licking and grooming during the first week of life showed decreased histone acetylation and increased DNA methylation of a neuron-specific promoter of the glucocorticoid receptor gene (Weaver et al., 2004). The expression of this gene is then reduced, the number of glucocorticoid receptors in the brain is decreased, and the animals show a higher hormonal response to stress throughout their life. The effects of maternal care on stress hormone responses and behaviour in the offspring can be eliminated in adulthood by pharmacological treatment (HDAC inhibitor trichostatin A, TSA) or dietary amino acid supplementation (methyl donor L-methionine), treatments that influence histone acetylation, DNA methylation, and expression of the glucocorticoid receptor gene (Weaver et al., 2004; Weaver et al., 2005). This series of experiments shows that histone acetylation and DNA methylation of the glucocorticoid receptor gene promoter is a necessary link in the process leading to the long-term physiological and behavioral sequelae of poor maternal care. This points to a possible molecular target for treatments that may reverse or ameliorate the traces of childhood maltreatment.
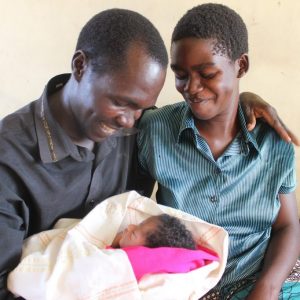
Several studies have attempted to determine to what extent the findings from model animals are transferable to humans. Examination of post-mortem brain tissue from healthy human subjects found that the human equivalent of the glucocorticoid receptor gene promoter (NR3C1 exon 1F promoter) is also unique to the individual (Turner, Pelascini, Macedo, & Muller, 2008). A similar study examining newborns showed that methylation of the glucocorticoid receptor gene promoter maybe an early epigenetic marker of maternal mood and risk of increased hormonal responses to stress in infants 3 months of age (Oberlander et al., 2008). Although further studies are required to examine the functional consequence of this DNA methylation, these findings are consistent with our studies in the neonate and adult offspring of low licking and grooming mothers that show increased DNA methylation of the promoter of the glucocorticoid receptor gene, decreased glucocorticoid receptor gene expression, and increased hormonal responses to stress (Weaver et al., 2004). Examination of brain tissue from suicide victims found that the human glucocorticoid receptor gene promoter is also more methylated in the brains of individuals who had experienced maltreatment during childhood (McGowan et al., 2009). These finding suggests that DNA methylation mediates the effects of early environment in both rodents and humans and points to the possibility of new therapeutic approaches stemming from translational epigenetic research. Indeed, similar processes at comparable epigenetic labile regions could explain why the adult offspring of high and low licking/grooming mothers exhibit widespread differences in hippocampal gene expression and cognitive function (Weaver, Meaney, & Szyf, 2006).
However, this type of research is limited by the inaccessibility of human brain samples. The translational potential of this finding would be greatly enhanced if the relevant epigenetic modification can be measured in an accessible tissue. Examination of blood samples from adult patients with bipolar disorder, who also retrospectively reported on their experiences of childhood abuse and neglect, found that the degree of DNA methylation of the human glucocorticoid receptor gene promoter was strongly positively related to the reported experience of childhood maltreatment decades earlier. For a relationship between a molecular measure and reported historical exposure, the effects size is extraordinarily large. This opens a range of new possibilities: given the large effect size and consistency of this association, measurement of the GR promoter methylation may effectively become a blood test measuring the physiological traces left on the genome by early experiences. Although this blood test cannot replace current methods of diagnosis, this unique and addition information adds to our knowledge of how disease may arise and be manifested throughout life. Near-future research will examine whether this measure adds value over and above simple reporting of early adversities when it comes to predicting important outcomes, such as response to treatment or suicide.
Child nutrition and the epigenome
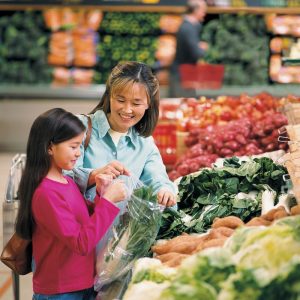
The old adage “you are what you eat” might be true on more than just a physical level: The food you choose (and even what your parents and grandparents chose) is reflected in your own personal development and risk for disease in adult life (Wells, 2003). Nutrients can reverse or change DNA methylation and histone modifications, thereby modifying the expression of critical genes associated with physiologic and pathologic processes, including embryonic development, aging, and carcinogenesis. It appears that nutrients can influence the epigenome either by directly inhibiting enzymes that catalyze DNA methylation or histone modifications, or by altering the availability of substrates necessary for those enzymatic reactions. For example, rat mothers fed a diet low in methyl group donors during pregnancy produce offspring with reduced DNMT-1 expression, decreased DNA methylation, and increased histone acetylation at promoter regions of specific genes, including the glucocorticoid receptor, and increased gene expression in the liver of juvenile offspring (Lillycrop, Phillips, Jackson, Hanson, & Burdge, 2005) and adult offspring (Lillycrop et al., 2007). These data suggest that early life nutrition has the potential to influence epigenetic programming in the brain not only during early development but also in adult life, thereby modulating health throughout life. In this regard, nutritional epigenetics has been viewed as an attractive tool to prevent pediatric developmental diseases and cancer, as well as to delay aging-associated processes.
The best evidence relating to the impact of adverse environmental conditions development and health comes from studies of the children of women who were pregnant during two civilian famines of World War II: the Siege of Leningrad (1941–44) (Bateson, 2001) and the Dutch Hunger Winter (1944–1945) (Stanner et al., 1997). In the Netherlands famine, women who were previously well nourished were subjected to low caloric intake and associated environmental stressors. Women who endured the famine in the late stages of pregnancy gave birth to smaller babies (Lumey & Stein, 1997) and these children had an increased risk of insulin resistance later in life (Painter, Roseboom, & Bleker, 2005). In addition, offspring who were starved prenatally later experienced impaired glucose tolerance in adulthood, even when food was more abundant (Stanner et al., 1997). Famine exposure at various stages of gestation was associated with a wide range of risks such as increased obesity, higher rates of coronary heart disease, and lower birth weight (Lumey & Stein, 1997). Interestingly, when examined 60 years later, people exposed to famine prenatally showed reduced DNA methylation compared with their unexposed same-sex siblings (Heijmans et al., 2008).
Epigenetic regulation of learning and memory
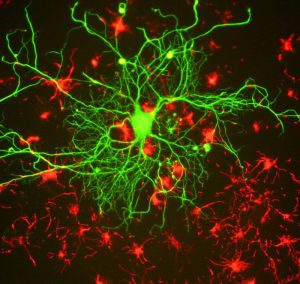
Memories are recollections of actual events stored within our brains. But how is our brain able to form and store these memories? Epigenetic mechanisms influence genomic activities in the brain to produce long-term changes in synaptic signaling, organization, and morphology, which in turn support learning and memory (Day & Sweatt, 2011).
Neuronal activity in the hippocampus of mice is associated with changes in DNA methylation (Guo et al., 2011), and disruption to genes encoding the DNA methylation machinery cause learning and memory impairments (Feng et al., 2010). DNA methylation has also been implicated in the maintenance of long-term memories, as pharmacological inhibition of DNA methylation and impaired memory (Day & Sweatt, 2011; Miller et al., 2010). These findings indicate the importance of DNA methylation in mediating synaptic plasticity and cognitive functions, both of which are disturbed in psychological illness.
Changes in histone modifications can also influence long-term memory formation by altering chromatin accessibility and the expression of genes relevant to learning and memory. Memory formation and the associated enhancements in synaptic transmission are accompanied by increases in histone acetylation (Guan et al., 2002) and alterations in histone methylation (Schaefer et al., 2009), which promote gene expression. Conversely, a neuronal increase in histone deacetylase activity, which promotes gene silencing, results in reduced synaptic plasticity and impairs memory (Guan et al., 2009). Pharmacological inhibition of histone deacetylases augments memory formation (Guan et al., 2009; Levenson et al., 2004), further suggesting that histone (de)acetylation regulates this process.
In humans genetic defects in genes encoding the DNA methylation and chromatin machinery exhibit profound effects on cognitive function and mental health (Jiang, Bressler, & Beaudet, 2004). The two best-characterized examples are Rett syndrome (Amir et al., 1999) and Rubinstein-Taybi syndrome (RTS) (Alarcon et al., 2004), which are profound intellectual disability disorders. Both MECP2 and CBP are highly expressed in neurons and are involved in regulating neural gene expression (Chen et al., 2003; Martinowich et al., 2003).
Rett syndrome patients have a mutation in their DNA sequence in a gene called MECP2. MECP2 plays many important roles within the cell: One of these roles is to read the DNA sequence, checking for DNA methylation, and to bind to areas that contain methylation, thereby preventing the wrong proteins from being present. Other roles for MECP2 include promoting the presence of particular, necessary, proteins, ensuring that DNA is packaged properly within the cell and assisting with the production of proteins. MECP2 function also influences gene expression that supports dendritic and synaptic development and hippocampus-dependent memory (Li, Zhong, Chau, Williams, & Chang, 2011; Skene et al., 2010). Mice with altered MECP2 expression exhibit genome-wide increases in histone acetylation, neuron cell death, increased anxiety, cognitive deficits, and social withdrawal (Shahbazian et al., 2002). These findings support a model in which DNA methylation and MECP2 constitute a cell-specific epigenetic mechanism for regulation of histone modification and gene expression, which may be disrupted in Rett syndrome.
RTS patients have a mutation in their DNA sequence in a gene called CBP. One of these roles of CBP is to bind to specific histones and promote histone acetylation, thereby promoting gene expression. Consistent with this function, RTS patients exhibit a genome-wide decrease in histone acetylation and cognitive dysfunction in adulthood (Kalkhoven et al., 2003). The learning and memory deficits are attributed to disrupted neural plasticity (Korzus, Rosenfeld, & Mayford, 2004). Similar to RTS in humans, mice with a mutation of CBP perform poorly in cognitive tasks and show decreased genome-wide histone acetylation (for review, see Josselyn, 2005). In the mouse brain CBP was found to act as an epigenetic switch to promote the birth of new neurons in the brain. Interestingly, this epigenetic mechanism is disrupted in the fetal brains of mice with a mutation of CBP, which, as pups, exhibit early behavioral deficits following removal and separation from their mother (Wang et al., 2010). These findings provide a novel mechanism whereby environmental cues, acting through histone modifying enzymes, can regulate epigenetic status and thereby directly promote neurogenesis, which regulates neurobehavioral development.
Together, these studies demonstrate that misregulation of epigenetic modifications and their regulatory enzymes is capable of orchestrating prominent deficits in neuronal plasticity and cognitive function. Knowledge from these studies may provide greater insight into other mental disorders such as depression and suicidal behaviors.
Epigenetic mechanisms in psychological disorders
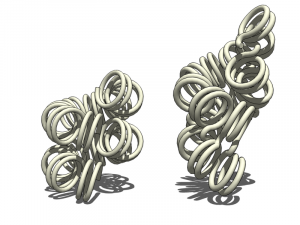
Epigenome-wide studies have identified several dozen sites with DNA methylation alterations in genes involved in brain development and neurotransmitter pathways, which had previously been associated with mental illness (Mill et al., 2008). These disorders are complex and typically start at a young age and cause lifelong disability. Often, limited benefits from treatment make these diseases some of the most burdensome disorders for individuals, families, and society. It has become evident that the efforts to identify the primary causes of complex psychiatric disorders may significantly benefit from studies linking environmental effects with changes observed within the individual cells.
Epigenetic events that alter chromatin structure to regulate programs of gene expression have been associated with depression-related behavior and action of antidepressant medications, with increasing evidence for similar mechanisms occurring in post-mortem brains of depressed individuals. In mice, social avoidance resulted in decreased expression of hippocampal genes important in mediating depressive responses (Tsankova et al., 2006). Similarly, chronic social defeat stress was found to decrease expression of genes implicated in normal emotion processing (Lutter et al., 2008). Consistent with these findings, levels of histone markers of increased gene expression were down regulated in human post-mortem brain samples from individuals with a history of clinical depression (Covington et al., 2009).
Administration of antidepressants increased histone markers of increased gene expression and reversed the gene repression induced by defeat stress (Lee, Wynder, Schmidt, McCafferty, & Shiekhattar, 2006; Tsankova et al., 2006; Wilkinson et al., 2009). These results provide support for the use of HDAC inhibitors against depression. Accordingly, several HDAC inhibitors have been found to exert antidepressant effects by each modifying distinct cellular targets (Cassel et al., 2006; Schroeder, Lin, Crusio, & Akbarian, 2007).
There is also increasing evidence that aberrant gene expression resulting from altered epigenetic regulation is associated with the pathophysiology of suicide (McGowan et al., 2008; Poulter et al., 2008). Thus, it is tempting to speculate that there is an epigenetically determined reduced capacity for gene expression, which is required for learning and memory, in the brains of suicide victims.
Epigenetic strategy to understanding gene-environment interactions
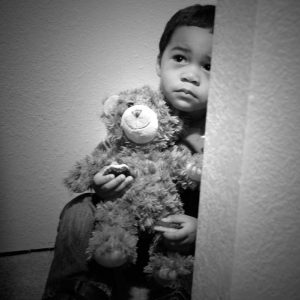
While the cellular and molecular mechanisms that influence on physical and mental health have long been a central focus of neuroscience, only in recent years has attention turned to the epigenetic mechanisms behind the dynamic changes in gene expression responsible for normal cognitive function and increased risk for mental illness. The links between early environment and epigenetic modifications suggest a mechanism underlying gene-environment interactions. Early environmental adversity alone is not a sufficient cause of mental illness, because many individuals with a history of severe childhood maltreatment or trauma remain healthy. It is increasingly becoming evident that inherited differences in the segments of specific genes may moderate the effects of adversity and determine who is sensitive and who is resilient through a gene-environment interplay. Genes such as the glucocorticoid receptor appear to moderate the effects of childhood adversity on mental illness. Remarkably, epigenetic DNA modifications have been identified that may underlie the long-lasting effects of environment on biological functions. This new epigenetic research is pointing to a new strategy to understanding gene-environment interactions.
The next decade of research will show if this potential can be exploited in the development of new therapeutic options that may alter the traces that early environment leaves on the genome. However, as discussed in this module, the epigenome is not static and can be molded by developmental signals, environmental perturbations, and disease states, which present an experimental challenge in the search for epigenetic risk factors in psychological disorders (Rakyan, Down, Balding, & Beck, 2011). The sample size and epigenomic assay required is dependent on the number of tissues affected, as well as the type and distribution of epigenetic modifications. The combination of genetic association maps studies with epigenome-wide developmental studies may help identify novel molecular mechanisms to explain features of inheritance of personality traits and transform our understanding of the biological basis of psychology. Importantly, these epigenetic studies may lead to identification of novel therapeutic targets and enable the development of improved strategies for early diagnosis, prevention, and better treatment of psychological and behavioral disorders.
A "Big Picture" Insight into Epi-Genetics
Epigentics is a content area where students sometimes feel apprehensive, especially if they do not have a background in biology. This course will teach you all required biology content expected for this class. To help get you excited about the importance of this research field, please watch the following video. It provides insights into why it is so important for behavioural scientists to understand, at least on a surface level, about the field of epigenetics. After you watch it, please be sure to go through this module again. This video is not intended as a replacement for the content in this module, but rather is intended to serve as a big-picture framework for you to better understand the content in this module.
Check Your Knowledge
To help you with your studying, we’ve included some practice questions for this module. These questions do not necessarily address all content in this module. They are intended as practice, and you are responsible for all of the content in this module even if there is no associated practice question. To promote deeper engagement with the material, we encourage you to create some questions of your own for your practice. You can then also return to these self-generated questions later in the course to test yourself.
Vocabulary
- DNA methylation
- Covalent modifications of mammalian DNA occurring via the methylation of cytosine, typically in the context of the CpG dinucleotide.
- DNA methyltransferases (DNMTs)
- Enzymes that establish and maintain DNA methylation using methyl-group donor compounds or cofactors. The main mammalian DNMTs are DNMT1, which maintains methylation state across DNA replication, and DNMT3a and DNMT3b, which perform de novo methylation.
- Epigenetics
- The study of heritable changes in gene expression or cellular phenotype caused by mechanisms other than changes in the underlying DNA sequence. Epigenetic marks include covalent DNA modifications and posttranslational histone modifications.
- Epigenome
- The genome-wide distribution of epigenetic marks.
- Gene
- A specific deoxyribonucleic acid (DNA) sequence that codes for a specific polypeptide or protein or an observable inherited trait.
- Genome-wide association study (GWAS)
- A study that maps DNA polymorphisms in affected individuals and controls matched for age, sex, and ethnic background with the aim of identifying causal genetic variants.
- Genotype
- The DNA content of a cell’s nucleus, whether a trait is externally observable or not.
- Histone acetyltransferases (HATs) and histone deacetylases (HDACs)
- HATs are enzymes that transfer acetyl groups to specific positions on histone tails, promoting an “open” chromatin state and transcriptional activation. HDACs remove these acetyl groups, resulting in a “closed” chromatin state and transcriptional repression.
- Histone modifications
- Posttranslational modifications of the N-terminal “tails” of histone proteins that serve as a major mode of epigenetic regulation. These modifications include acetylation, phosphorylation, methylation, sumoylation, ubiquitination, and ADP-ribosylation.
- Identical twins
- Two individual organisms that originated from the same zygote and therefore are genetically identical or very similar. The epigenetic profiling of identical twins discordant for disease is a unique experimental design as it eliminates the DNA sequence-, age-, and sex-differences from consideration.
- Phenotype
- The pattern of expression of the genotype or the magnitude or extent to which it is observably expressed—an observable characteristic or trait of an organism, such as its morphology, development, biochemical or physiological properties, or behavior.
References
- Adams, R. L., McKay, E. L., Craig, L. M., & Burdon, R. H. (1979). Mouse DNA methylase: methylation of native DNA. Biochimica et Biophysica Acta, 561(2), 345–357.
- Alarcon, J. M., Malleret, G., Touzani, K., Vronskaya, S., Ishii, S., Kandel, E. R., & Barco, A. (2004). Chromatin acetylation, memory, and LTP are impaired in CBP+/- mice: a model for the cognitive deficit in Rubinstein-Taybi syndrome and its amelioration. Neuron, 42(6), 947–959. doi: 10.1016/j.neuron.2004.05.021, S0896627304003022 [pii]
- Amir, R. E., Van den Veyver, I. B., Wan, M., Tran, C. Q., Francke, U., & Zoghbi, H. Y. (1999). Rett syndrome is caused by mutations in X-linked MECP2, encoding methyl-CpG-binding protein 2. Nature Genetics, 23(2), 185–188.
- Bateson, P. (2001). Fetal experience and good adult design. International Journal of Epidemiology, 30(5), 928–934.
- Bradshaw, A.D. (1965). Evolutionary significance of phenotypic plasticity in plants. Advances in Genetics, 13, 115–155.
- Caldji, C., Tannenbaum, B., Sharma, S., Francis, D., Plotsky, P. M., & Meaney, M. J. (1998). Maternal care during infancy regulates the development of neural systems mediating the expression of fearfulness in the rat. Proceedings of the National Academy of Sciences U S A, 95(9), 5335–5340.
- Cassel, S., Carouge, D., Gensburger, C., Anglard, P., Burgun, C., Dietrich, J. B., . . . Zwiller, J. (2006). Fluoxetine and cocaine induce the epigenetic factors MeCP2 and MBD1 in adult rat brain. Molecular Pharmacology, 70(2), 487–492. doi: 10.1124/mol.106.022301
- Chen, W. G., Chang, Q., Lin, Y., Meissner, A., West, A. E., Griffith, E. C., . . . Greenberg, M. E. (2003). Derepression of BDNF transcription involves calcium-dependent phosphorylation of MeCP2. Science, 302(5646), 885–889.
- Covington, H. E., 3rd, Maze, I., LaPlant, Q. C., Vialou, V. F., Ohnishi, Y. N., Berton, O., . . . Nestler, E. J. (2009). Antidepressant actions of histone deacetylase inhibitors. Journal of Neuroscience, 29(37), 11451–11460. doi: 10.1523/JNEUROSCI.1758-09.2009
- Davie, J. R., & Chadee, D. N. (1998). Regulation and regulatory parameters of histone modifications. *Journal of Cellular Biochemistry Suppl, 30–31 *, 203–213.
- Day, J. J., & Sweatt, J. D. (2011). Epigenetic mechanisms in cognition. Neuron, 70(5), 813–829. doi: 10.1016/j.neuron.2011.05.019
- Dick, D. M., Riley, B., & Kendler, K. S. (2010). Nature and nurture in neuropsychiatric genetics: where do we stand? Dialogues in Clinical Neuroscience, 12(1), 7–23.
- Dolinoy, D. C., Weidman, J. R., & Jirtle, R. L. (2007). Epigenetic gene regulation: linking early developmental environment to adult disease. Reproductive Toxicology, 23(3), 297–307. doi: S0890-6238(06)00197-3 [pii], 10.1016/j.reprotox.2006.08.012
- Feng, J., Chang, H., Li, E., & Fan, G. (2005). Dynamic expression of de novo DNA methyltransferases Dnmt3a and Dnmt3b in the central nervous system. Journal of Neuroscience Research, 79(6), 734–746. doi: 10.1002/jnr.20404
- Feng, J., Zhou, Y., Campbell, S. L., Le, T., Li, E., Sweatt, J. D., . . . Fan, G. (2010). Dnmt1 and Dnmt3a maintain DNA methylation and regulate synaptic function in adult forebrain neurons. Nature Neuroscience, 13(4), 423–430. doi: 10.1038/nn.2514
- Francis, D., Diorio, J., Liu, D., & Meaney, M. J. (1999). Nongenomic transmission across generations of maternal behavior and stress responses in the rat. Science, 286(5442), 1155–1158.
- Gershon, E. S., Alliey-Rodriguez, N., & Liu, C. (2011). After GWAS: searching for genetic risk for schizophrenia and bipolar disorder. American Journal of Psychiatry, 168(3), 253–256. doi: 10.1176/appi.ajp.2010.10091340
- Goll, M. G., & Bestor, T. H. (2005). Eukaryotic cytosine methyltransferases. Annual Review of Biochemistry, 74, 481–514. doi: 10.1146/annurev.biochem.74.010904.153721
- Goto, K., Numata, M., Komura, J. I., Ono, T., Bestor, T. H., & Kondo, H. (1994). Expression of DNA methyltransferase gene in mature and immature neurons as well as proliferating cells in mice. Differentiation, 56(1–2), 39–44.
- Gregg, C., Zhang, J., Weissbourd, B., Luo, S., Schroth, G. P., Haig, D., & Dulac, C. (2010). High-resolution analysis of parent-of-origin allelic expression in the mouse brain. Science, 329(5992), 643–648. doi: 10.1126/science.1190830
- Guan, J. S., Haggarty, S. J., Giacometti, E., Dannenberg, J. H., Joseph, N., Gao, J., . . . Tsai, L. H. (2009). HDAC2 negatively regulates memory formation and synaptic plasticity. Nature, 459(7243), 55-60. doi: 10.1038/nature07925
- Guan, Z., Giustetto, M., Lomvardas, S., Kim, J. H., Miniaci, M. C., Schwartz, J. H., . . . Kandel, E. R. (2002). Integration of long-term-memory-related synaptic plasticity involves bidirectional regulation of gene expression and chromatin structure. Cell, 111(4), 483–493.
- Guo, J. U., Ma, D. K., Mo, H., Ball, M. P., Jang, M. H., Bonaguidi, M. A., . . . Song, H. (2011). Neuronal activity modifies the DNA methylation landscape in the adult brain. Nature Neuroscience, 14(10), 1345–1351. doi: 10.1038/nn.2900
- Heijmans, B. T., Tobi, E. W., Stein, A. D., Putter, H., Blauw, G. J., Susser, E. S., . . . Lumey, L. H. (2008). Persistent epigenetic differences associated with prenatal exposure to famine in humans. Proceedings of the National Academy of Sciences U S A, 105(44), 17046–17049. doi: 0806560105 [pii]10.1073/pnas.0806560105
- Hong, L., Schroth, G. P., Matthews, H. R., Yau, P., & Bradbury, E. M. (1993). Studies of the DNA binding properties of histone H4 amino terminus. Thermal denaturation studies reveal that acetylation markedly reduces the binding constant of the H4 "tail" to DNA. Journal of Biological Chemistry, 268(1), 305–314.
- Jenuwein, T., & Allis, C. D. (2001). Translating the histone code. Science, 293(5532), 1074–1080. doi: 10.1126/Science.1063127293/5532/1074 [pii]
- Jiang, Y. H., Bressler, J., & Beaudet, A. L. (2004). Epigenetics and human disease. Annual Review of Genomics and Human Genetics, 5, 479–510. doi: 10.1146/annurev.genom.5.061903.180014
- Josselyn, S. A. (2005). What's right with my mouse model? New insights into the molecular and cellular basis of cognition from mouse models of Rubinstein-Taybi Syndrome. Learning & Memory, 12(2), 80–83. doi: 12/2/80 [pii]10.1101/lm.93505
- Kadonaga, J. T. (1998). Eukaryotic transcription: an interlaced network of transcription factors and chromatin-modifying machines. Cell, 92(3), 307–313.
- Kalkhoven, E., Roelfsema, J. H., Teunissen, H., den Boer, A., Ariyurek, Y., Zantema, A., . . . Peters, D. J. (2003). Loss of CBP acetyltransferase activity by PHD finger mutations in Rubinstein-Taybi syndrome. Human Molecular Genetics, 12(4), 441–450.
- Korzus, E., Rosenfeld, M. G., & Mayford, M. (2004). CBP histone acetyltransferase activity is a critical component of memory consolidation. Neuron, 42(6), 961–972. doi: 10.1016/j.neuron.2004.06.002S0896627304003526 [pii]
- Kuo, M. H., & Allis, C. D. (1998). Roles of histone acetyltransferases and deacetylases in gene regulation. Bioessays, 20(8), 615–626. doi: 10.1002/(SICI)1521–1878(199808)20:8<615::AID-BIES4>3.0.CO;2-H [pii] 10.1002/(SICI)1521-1878(199808)20:8<615::AID-BIES4>3.0.CO;2-H
- Law, J. A., & Jacobsen, S. E. (2010). Establishing, maintaining and modifying DNA methylation patterns in plants and animals. Nature Reviews Genetics, 11(3), 204–220. doi: nrg2719 [pii]10.1038/nrg2719
- Lee, M. G., Wynder, C., Schmidt, D. M., McCafferty, D. G., & Shiekhattar, R. (2006). Histone H3 lysine 4 demethylation is a target of nonselective antidepressive medications. Chemistry & Biology, 13(6), 563–567. doi: 10.1016/j.chembiol.2006.05.004
- Levenson, J. M., O'Riordan, K. J., Brown, K. D., Trinh, M. A., Molfese, D. L., & Sweatt, J. D. (2004). Regulation of histone acetylation during memory formation in the hippocampus. Journal of Biological Chemistry, 279(39), 40545–40559.
- Li, H., Zhong, X., Chau, K. F., Williams, E. C., & Chang, Q. (2011). Loss of activity-induced phosphorylation of MeCP2 enhances synaptogenesis, LTP and spatial memory. Nature Neuroscience, 14(8), 1001–1008. doi: 10.1038/nn.2866
- Lillycrop, K. A., Phillips, E. S., Jackson, A. A., Hanson, M. A., & Burdge, G. C. (2005). Dietary protein restriction of pregnant rats induces and folic acid supplementation prevents epigenetic modification of hepatic gene expression in the offspring. Journal of Nutrition, 135(6), 1382–1386. doi: 135/6/1382 [pii]
- Lillycrop, K. A., Slater-Jefferies, J. L., Hanson, M. A., Godfrey, K. M., Jackson, A. A., & Burdge, G. C. (2007). Induction of altered epigenetic regulation of the hepatic glucocorticoid receptor in the offspring of rats fed a protein-restricted diet during pregnancy suggests that reduced DNA methyltransferase-1 expression is involved in impaired DNA methylation and changes in histone modifications. British Journal of Nutrition, 97(6), 1064–1073. doi: S000711450769196X [pii]10.1017/S000711450769196X
- Liu, D., Diorio, J., Tannenbaum, B., Caldji, C., Francis, D., Freedman, A., . . . Meaney, M. J. (1997). Maternal care, hippocampal glucocorticoid receptors, and hypothalamic- pituitary-adrenal responses to stress [see comments]. Science, 277(5332), 1659–1662.
- Lumey, L. H., & Stein, A. D. (1997). Offspring birth weights after maternal intrauterine undernutrition: a comparison within sibships. American Journal of Epidemiology, 146(10), 810–819.
- Lutter, M., Krishnan, V., Russo, S. J., Jung, S., McClung, C. A., & Nestler, E. J. (2008). Orexin signaling mediates the antidepressant-like effect of calorie restriction. Journal of Neuroscience, 28(12), 3071–3075. doi: 10.1523/JNEUROSCI.5584-07.2008
- Martinowich, K., Hattori, D., Wu, H., Fouse, S., He, F., Hu, Y., . . . Sun, Y. E. (2003). DNA methylation-related chromatin remodeling in activity-dependent BDNF gene regulation. Science, 302(5646), 890–893.
- McGowan, P. O., Sasaki, A., D'Alessio, A. C., Dymov, S., Labonte, B., Szyf, M., . . . Meaney, M. J. (2009). Epigenetic regulation of the glucocorticoid receptor in human brain associates with childhood abuse. Nature Neuroscience, 12(3), 342–348. doi: nn.2270 [pii]10.1038/nn.2270
- McGowan, P. O., Sasaki, A., Huang, T. C., Unterberger, A., Suderman, M., Ernst, C., . . . Szyf, M. (2008). Promoter-wide hypermethylation of the ribosomal RNA gene promoter in the suicide brain. PLoS ONE, 3(5), e2085. doi: 10.1371/journal.pone.0002085
- Mill, J., Tang, T., Kaminsky, Z., Khare, T., Yazdanpanah, S., Bouchard, L., . . . Petronis, A. (2008). Epigenomic profiling reveals DNA-methylation changes associated with major psychosis. American Journal of Human Genetics, 82(3), 696–711. doi: 10.1016/j.ajhg.2008.01.008
- Miller, C. A., Gavin, C. F., White, J. A., Parrish, R. R., Honasoge, A., Yancey, C. R., . . . Sweatt, J. D. (2010). Cortical DNA methylation maintains remote memory. Nature Neuroscience, 13(6), 664–666. doi: 10.1038/nn.2560
- Myers, M. M., Brunelli, S. A., Shair, H. N., Squire, J. M., & Hofer, M. A. (1989). Relationships between maternal behavior of SHR and WKY dams and adult blood pressures of cross-fostered F1 pups. Developmental Psychobiology, 22(1), 55–67.
- Oberlander, T. F., Weinberg, J., Papsdorf, M., Grunau, R., Misri, S., & Devlin, A. M. (2008). Prenatal exposure to maternal depression, neonatal methylation of human glucocorticoid receptor gene (NR3C1) and infant cortisol stress responses. Epigenetics, 3(2), 97–106. doi: 6034 [pii]
- Ooi, S. K., O'Donnell, A. H., & Bestor, T. H. (2009). Mammalian cytosine methylation at a glance. Journal of Cell Science, 122(Pt 16), 2787–2791. doi: 122/16/2787 [pii]10.1242/jcs.015123
- Painter, R. C., Roseboom, T. J., & Bleker, O. P. (2005). Prenatal exposure to the Dutch famine and disease in later life: an overview. Reproductive Toxicology, 20(3), 345–352. doi: S0890-6238(05)00088-2 [pii]10.1016/j. Reproductive Toxicology.2005.04.005
- Petronis, A. (2010). Epigenetics as a unifying principle in the aetiology of complex traits and diseases. Nature, 465(7299), 721–727. doi: 10.1038/nature09230
- Poulter, M. O., Du, L., Weaver, I. C., Palkovits, M., Faludi, G., Merali, Z., . . . Anisman, H. (2008). GABAA receptor promoter hypermethylation in suicide brain: implications for the involvement of epigenetic processes. Biological Psychiatry, 64(8), 645–652. doi: 10.1016/j.biopsych.2008.05.028
- Rakyan, V. K., Down, T. A., Balding, D. J., & Beck, S. (2011). Epigenome-wide association studies for common human diseases. Nature Reviews Genetics, 12(8), 529–541. doi: 10.1038/nrg3000
- Razin, A. (1998). CpG methylation, chromatin structure and gene silencing-a three-way connection. European Molecular Biology Organization, 17(17), 4905–4908.
- Schaefer, A., Sampath, S. C., Intrator, A., Min, A., Gertler, T. S., Surmeier, D. J., . . . Greengard, P. (2009). Control of cognition and adaptive behavior by the GLP/G9a epigenetic suppressor complex. Neuron, 64(5), 678–691. doi: 10.1016/j.neuron.2009.11.019
- Schroeder, F. A., Lin, C. L., Crusio, W. E., & Akbarian, S. (2007). Antidepressant-like effects of the histone deacetylase inhibitor, sodium butyrate, in the mouse. Biological Psychiatry, 62(1), 55-64. doi: 10.1016/j.biopsych.2006.06.036
- Sealy, L., & Chalkley, R. (1978). DNA associated with hyperacetylated histone is preferentially digested by DNase I. Nucleic Acids Research, 5(6), 1863–1876.
- Shahbazian, M., Young, J., Yuva-Paylor, L., Spencer, C., Antalffy, B., Noebels, J., . . . Zoghbi, H. (2002). Mice with truncated MeCP2 recapitulate many Rett syndrome features and display hyperacetylation of histone H3. Neuron, 35(2), 243–254.
- Skene, P. J., Illingworth, R. S., Webb, S., Kerr, A. R., James, K. D., Turner, D. J., . . . Bird, A. P. (2010). Neuronal MeCP2 is expressed at near histone-octamer levels and globally alters the chromatin state. Molecular Cell, 37(4), 457–468. doi: 10.1016/j.molcel.2010.01.030
- Stanner, S. A., Bulmer, K., Andres, C., Lantseva, O. E., Borodina, V., Poteen, V. V., & Yudkin, J. S. (1997). Does malnutrition in utero determine diabetes and coronary heart disease in adulthood? Results from the Leningrad siege study, a cross sectional study. British Medical Journal, 315(7119), 1342–1348.
- Stern, J. M. (1997). Offspring-induced nurturance: animal-human parallels. Developmental Psychobiololgy, 31(1), 19–37.
- Sutter, D., Doerfler, W., 1980. Methylation of integrated adenovirus type 12 DNA sequences in transformed cells is inversely correlated with viral gene expression. Proceedings of the National Academy of Sciences U S A. 77, 253–256.
- Suzuki, M. M., & Bird, A. (2008). DNA methylation landscapes: provocative insights from epigenomics. Nature Reviews Genetics, 9(6), 465–476. doi: nrg2341 [pii]10.1038/nrg2341
- Tsankova, N. M., Berton, O., Renthal, W., Kumar, A., Neve, R. L., & Nestler, E. J. (2006). Sustained hippocampal chromatin regulation in a mouse model of depression and antidepressant action. Nature Neuroscience. 9(4): 519–525. doi:10.1038/nn1659
- Turner, J. D., Pelascini, L. P., Macedo, J. A., & Muller, C. P. (2008). Highly individual methylation patterns of alternative glucocorticoid receptor promoters suggest individualized epigenetic regulatory mechanisms. Nucleic Acids Research, 36(22), 7207–7218. doi: gkn897 [pii] 10.1093/nar/gkn897
- Vardimon, L., Kressmann, A., Cedar, H., Maechler, M., Doerfler, W., 1982. Expression of a cloned adenovirus gene is inhibited by in vitro methylation. Proceedings of the National Academy of Sciences U S A. 79, 1073–1077.
- Waddington, C. H. (1942). Epigenotype. Endeavour(1), 18–21.
- Wade, P. A., Pruss, D., & Wolffe, A. P. (1997). Histone acetylation: chromatin in action. Trends in Biochemical Sciences, 22(4), 128–132. doi: S0968000497010165 [pii]
- Wang, J., Weaver, I. C., Gauthier-Fisher, A., Wang, H., He, L., Yeomans, J., . . . Miller, F. D. (2010). CBP histone acetyltransferase activity regulates embryonic neural differentiation in the normal and Rubinstein-Taybi syndrome brain. Developmental Cell, 18(1), 114–125. doi: 10.1016/j.devcel.2009.10.023
- Weaver, I. C., Cervoni, N., Champagne, F. A., D'Alessio, A. C., Sharma, S., Seckl, J. R., . . . Meaney, M. J. (2004). Epigenetic programming by maternal behavior. Nature Neuroscience, 7(8), 847–854. doi: 10.1038/nn1276
- Weaver, I. C., Champagne, F. A., Brown, S. E., Dymov, S., Sharma, S., Meaney, M. J., & Szyf, M. (2005). Reversal of maternal programming of stress responses in adult offspring through methyl supplementation: altering epigenetic marking later in life. Journal of Neuroscience, 25(47), 11045–11054. doi: 10.1523/JNEUROSCI.3652-05.2005
- Weaver, I. C., Meaney, M. J., & Szyf, M. (2006). Maternal care effects on the hippocampal transcriptome and anxiety-mediated behaviors in the offspring that are reversible in adulthood. Proceedings of the National Academy of Sciences U S A, 103(9), 3480–3485. doi: 10.1073/pnas.0507526103
- Wells, J. C. (2003). The thrifty phenotype hypothesis: thrifty offspring or thrifty mother? Journal of Theoretical Biology, 221(1), 143–161.
- Wilkinson, M. B., Xiao, G., Kumar, A., LaPlant, Q., Renthal, W., Sikder, D., . . . Nestler, E. J. (2009). Imipramine treatment and resiliency exhibit similar chromatin regulation in the mouse nucleus accumbens in depression models. Journal of Neuroscience, 29(24), 7820–7832. doi: 10.1523/JNEUROSCI.0932-09.2009
- Wolffe, A. P., & Matzke, M. A. (1999). Epigenetics: regulation through repression. Science, 286(5439), 481–486.
How to cite this Chapter using APA Style:
Weaver, I. (2019). Epigenetics in psychology. Adapted for use by Queen's University. Original chapter in R. Biswas-Diener & E. Diener (Eds), Noba textbook series: Psychology.Champaign, IL: DEF publishers. Retrieved from http://noba.to/37p5cb8v
Copyright and Acknowledgment:
This material is licensed under the Creative Commons Attribution-NonCommercial-ShareAlike 4.0 International License. To view a copy of this license, visit: http://creativecommons.org/licenses/by-nc-sa/4.0/deed.en_US.
This material is attributed to the Diener Education Fund (copyright © 2018) and can be accessed via this link: http://noba.to/37p5cb8v.
Additional information about the Diener Education Fund (DEF) can be accessed here.
Original chapter by Ian Weaver adapted by Queen's University Psychology Department
This Open Access chapter was originally written for the NOBA project. Information on the NOBA project can be found below.
Early life experiences exert a profound and long-lasting influence on physical and mental health throughout life. The efforts to identify the primary causes of this have significantly benefited from studies of the epigenome—a dynamic layer of information associated with DNA that differs between individuals and can be altered through various experiences and environments. The epigenome has been heralded as a key “missing piece” of the etiological puzzle for understanding how development of psychological disorders may be influenced by the surrounding environment, in concordance with the genome. Understanding the mechanisms involved in the initiation, maintenance, and heritability of epigenetic states is thus an important aspect of research in current biology, particularly in the study of learning and memory, emotion, and social behavior in humans. Moreover, epigenetics in psychology provides a framework for understanding how the expression of genes is influenced by experiences and the environment to produce individual differences in behavior, cognition, personality, and mental health. In this module, we survey recent developments revealing epigenetic aspects of mental health and review some of the challenges of epigenetic approaches in psychology to help explain how nurture shapes nature.
Learning Objectives
- Explain what the term epigenetics means and the molecular machinery involved.
- Name and discuss important neural and developmental pathways that are regulated by epigenetic factors, and provide examples of epigenetic effects on personality traits and cognitive behavior.
- Understand how misregulation of epigenetic mechanisms can lead to disease states, and be able to discuss examples.
- Recognize how epigenetic machinery can be targets for therapeutic agents, and discuss examples.
Introduction
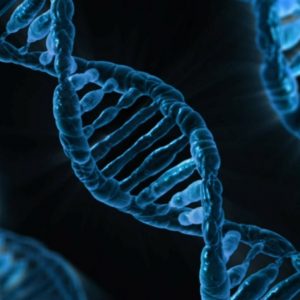
Early childhood is not only a period of physical growth; it is also a time of mental development related to changes in the anatomy, physiology, and chemistry of the nervous system that influence mental health throughout life. Cognitive abilities associated with learning and memory, reasoning, problem solving, and developing relationships continue to emerge during childhood. Brain development is more rapid during this critical or sensitive period than at any other, with more than 700 neural connections created each second. Herein, complex gene–environment interactions (or genotype–environment interactions, G×E) serve to increase the number of possible contacts between neurons, as they hone their adult synaptic properties and excitability. Many weak connections form to different neuronal targets; subsequently, they undergo remodeling in which most connections vanish and a few stable connections remain. These structural changes (or plasticity) may be crucial for the development of mature neural networks that support emotional, cognitive, and social behavior. The generation of different morphology, physiology, and behavioral outcomes from a single genome in response to changes in the environment forms the basis for “phenotypic plasticity,” which is fundamental to the way organisms cope with environmental variation, navigate the present world, and solve future problems.
The challenge for psychology has been to integrate findings from genetics and environmental (social, biological, chemical) factors, including the quality of infant–mother attachments, into the study of personality and our understanding of the emergence of mental illness. These studies have demonstrated that common DNA sequence variation and rare mutations account for only a small fraction (1%–2%) of the total risk for inheritance of personality traits and mental disorders (Dick, Riley, & Kendler, 2010; Gershon, Alliey-Rodriguez, & Liu, 2011). Additionally, studies that have attempted to examine the mechanisms and conditions under which DNA sequence variation influences brain development and function have been confounded by complex cause-and-effect relationships (Petronis, 2010). The large unaccounted heritability of personality traits and mental health suggests that additional molecular and cellular mechanisms are involved.
Epigenetics has the potential to provide answers to these important questions and refers to the transmission of phenotype in terms of gene expression in the absence of changes in DNA sequence—hence the name epi- (Greek: επί- over, above) genetics (Waddington, 1942; Wolffe & Matzke, 1999). The advent of high-throughput techniques such as sequencing-based approaches to study the distributions of regulators of gene expression throughout the genome led to the collective description of the “epigenome.” In contrast to the genome sequence, which is static and the same in almost all cells, the epigenome is highly dynamic, differing among cell types, tissues, and brain regions (Gregg et al., 2010). Recent studies have provided insights into epigenetic regulation of developmental pathways in response to a range of external environmental factors (Dolinoy, Weidman, & Jirtle, 2007). These environmental factors during early childhood and adolescence can cause changes in expression of genes conferring risk of mental health and chronic physical conditions. Thus, the examination of genetic–epigenetic–environment interactions from a developmental perspective may determine the nature of gene misregulation in psychological disorders.
This module will provide an overview of the main components of the epigenome and review themes in recent epigenetic research that have relevance for psychology, to form the biological basis for the interplay between environmental signals and the genome in the regulation of individual differences in physiology, emotion, cognition, and behavior.
Molecular control of gene expression: the dynamic epigenome
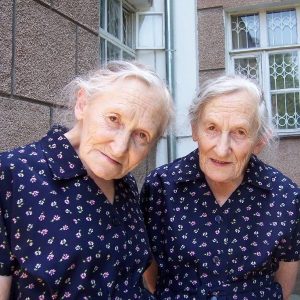
Almost all the cells in our body are genetically identical, yet our body generates many different cell types, organized into different tissues and organs, and expresses different proteins. Within each type of mammalian cell, about 2 meters of genomic DNA is divided into nuclear chromosomes. Yet the nucleus of a human cell, which contains the chromosomes, is only about 2 μm in diameter. To achieve this 1,000,000-fold compaction, DNA is wrapped around a group of 8 proteins called histones. This combination of DNA and histone proteins forms a special structure called a “nucleosome,” the basic unit of chromatin, which represents a structural solution for maintaining and accessing the tightly compacted genome. These factors alter the likelihood that a gene will be expressed or silenced. Cellular functions such as gene expression, DNA replication, and the generation of specific cell types are therefore influenced by distinct patterns of chromatin structure, involving covalent modification of both histones (Kadonaga, 1998) and DNA (Razin, 1998).
Importantly, epigenetic variation also emerges across the lifespan. For example, although identical twins share a common genotype and are genetically identical and epigenetically similar when they are young, as they age they become more dissimilar in their epigenetic patterns and often display behavioral, personality, or even physical differences, and have different risk levels for serious illness. Thus, understanding the structure of the nucleosome is key to understanding the precise and stable control of gene expression and regulation, providing a molecular interface between genes and environmentally induced changes in cellular activity.
The primary epigenetic mark: DNA modification
DNA methylation is the best-understood epigenetic modification influencing gene expression. DNA is composed of four types of naturally occurring nitrogenous bases: adenine (A), thymine (T), guanine (G), and cytosine (C). In mammalian genomes, DNA methylation occurs primarily at cytosine residues in the context of cytosines that are followed by guanines (CpG dinucleotides), to form 5-methylcytosine in a cell-specific pattern (Goll & Bestor, 2005; Law & Jacobsen, 2010; Suzuki & Bird, 2008). The enzymes that perform DNA methylation are called DNA methyltransferases (DNMTs), which catalyze the transfer of a methyl group to the cytosine (Adams, McKay, Craig, & Burdon, 1979). These enzymes are all expressed in the central nervous system and are dynamically regulated during development (Feng, Chang, Li, & Fan, 2005; Goto et al., 1994). The effect of DNA methylation on gene function varies depending on the period of development during which the methylation occurs and location of the methylated cytosine. Methylation of DNA in gene regulatory regions (promoter and enhancer regions) usually results in gene silencing and reduced gene expression (Ooi, O’Donnell, & Bestor, 2009; Suzuki & Bird, 2008; Sutter and Doerfler, 1980; Vardimon et al., 1982). This is a powerful regulatory mechanism that ensures that genes are expressed only when needed. Thus DNA methylation may broadly impact human brain development, and age-related misregulation of DNA methylation is associated with the molecular pathogenesis of neurodevelopmental disorders.
Histone modification and the histone code
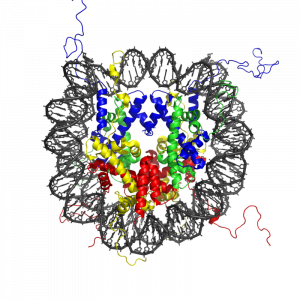
The modification of histone proteins comprises an important epigenetic mark related to gene expression. One of the most thoroughly studied modifications is histone acetylation, which is associated with gene activation and increased gene expression (Wade, Pruss, & Wolffe, 1997). Acetylation on histone tails is mediated by the opposing enzymatic activities of histone acetyltransferases (HATs) and histone deacetylases (HDACs) (Kuo & Allis, 1998). For example, acetylation of histone in gene regulatory regions by HAT enzymes is generally associated with DNA demethylation, gene activation, and increased gene expression (Hong, Schroth, Matthews, Yau, & Bradbury, 1993; Sealy & Chalkley, 1978). On the other hand, removal of the acetyl group (deacetylation) by HDAC enzymes is generally associated with DNA methylation, gene silencing, and decreased gene expression (Davie & Chadee, 1998). The relationship between patterns of histone modifications and gene activity provides evidence for the existence of a “histone code” for determining cell-specific gene expression programs (Jenuwein & Allis, 2001). Interestingly, recent research using animal models has demonstrated that histone modifications and DNA methylation of certain genes mediates the long-term behavioral effects of the level of care experienced during infancy.
Early childhood experience
The development of an individual is an active process of adaptation that occurs within a social and economic context. For example, the closeness or degree of positive attachment of the parent (typically mother)–infant bond and parental investment (including nutrient supply provided by the parent) that define early childhood experience also program the development of individual differences in stress responses in the brain, which then affect memory, attention, and emotion. In terms of evolution, this process provides the offspring with the ability to physiologically adjust gene expression profiles contributing to the organization and function of neural circuits and molecular pathways that support (1) biological defensive systems for survival (e.g., stress resilience), (2) reproductive success to promote establishment and persistence in the present environment, and (3) adequate parenting in the next generation (Bradshaw, 1965).
Parental investment and programming of stress responses in the offspring
The most comprehensive study to date of variations in parental investment and epigenetic inheritance in mammals is that of the maternally transmitted responses to stress in rats. In rat pups, maternal nurturing (licking and grooming) during the first week of life is associated with long-term programming of individual differences in stress responsiveness, emotionality, cognitive performance, and reproductive behavior (Caldji et al., 1998; Francis, Diorio, Liu, & Meaney, 1999; Liu et al., 1997; Myers, Brunelli, Shair, Squire, & Hofer, 1989; Stern, 1997). In adulthood, the offspring of mothers that exhibit increased levels of pup licking and grooming over the first week of life show increased expression of the glucocorticoid receptor in the hippocampus (a brain structure associated with stress responsivity as well as learning and memory) and a lower hormonal response to stress compared with adult animals reared by low licking and grooming mothers (Francis et al., 1999; Liu et al., 1997). Moreover, rat pups that received low levels of maternal licking and grooming during the first week of life showed decreased histone acetylation and increased DNA methylation of a neuron-specific promoter of the glucocorticoid receptor gene (Weaver et al., 2004). The expression of this gene is then reduced, the number of glucocorticoid receptors in the brain is decreased, and the animals show a higher hormonal response to stress throughout their life. The effects of maternal care on stress hormone responses and behaviour in the offspring can be eliminated in adulthood by pharmacological treatment (HDAC inhibitor trichostatin A, TSA) or dietary amino acid supplementation (methyl donor L-methionine), treatments that influence histone acetylation, DNA methylation, and expression of the glucocorticoid receptor gene (Weaver et al., 2004; Weaver et al., 2005). This series of experiments shows that histone acetylation and DNA methylation of the glucocorticoid receptor gene promoter is a necessary link in the process leading to the long-term physiological and behavioral sequelae of poor maternal care. This points to a possible molecular target for treatments that may reverse or ameliorate the traces of childhood maltreatment.
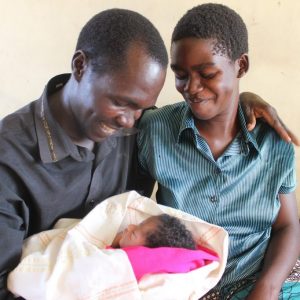
Several studies have attempted to determine to what extent the findings from model animals are transferable to humans. Examination of post-mortem brain tissue from healthy human subjects found that the human equivalent of the glucocorticoid receptor gene promoter (NR3C1 exon 1F promoter) is also unique to the individual (Turner, Pelascini, Macedo, & Muller, 2008). A similar study examining newborns showed that methylation of the glucocorticoid receptor gene promoter maybe an early epigenetic marker of maternal mood and risk of increased hormonal responses to stress in infants 3 months of age (Oberlander et al., 2008). Although further studies are required to examine the functional consequence of this DNA methylation, these findings are consistent with our studies in the neonate and adult offspring of low licking and grooming mothers that show increased DNA methylation of the promoter of the glucocorticoid receptor gene, decreased glucocorticoid receptor gene expression, and increased hormonal responses to stress (Weaver et al., 2004). Examination of brain tissue from suicide victims found that the human glucocorticoid receptor gene promoter is also more methylated in the brains of individuals who had experienced maltreatment during childhood (McGowan et al., 2009). These finding suggests that DNA methylation mediates the effects of early environment in both rodents and humans and points to the possibility of new therapeutic approaches stemming from translational epigenetic research. Indeed, similar processes at comparable epigenetic labile regions could explain why the adult offspring of high and low licking/grooming mothers exhibit widespread differences in hippocampal gene expression and cognitive function (Weaver, Meaney, & Szyf, 2006).
However, this type of research is limited by the inaccessibility of human brain samples. The translational potential of this finding would be greatly enhanced if the relevant epigenetic modification can be measured in an accessible tissue. Examination of blood samples from adult patients with bipolar disorder, who also retrospectively reported on their experiences of childhood abuse and neglect, found that the degree of DNA methylation of the human glucocorticoid receptor gene promoter was strongly positively related to the reported experience of childhood maltreatment decades earlier. For a relationship between a molecular measure and reported historical exposure, the effects size is extraordinarily large. This opens a range of new possibilities: given the large effect size and consistency of this association, measurement of the GR promoter methylation may effectively become a blood test measuring the physiological traces left on the genome by early experiences. Although this blood test cannot replace current methods of diagnosis, this unique and addition information adds to our knowledge of how disease may arise and be manifested throughout life. Near-future research will examine whether this measure adds value over and above simple reporting of early adversities when it comes to predicting important outcomes, such as response to treatment or suicide.
Child nutrition and the epigenome
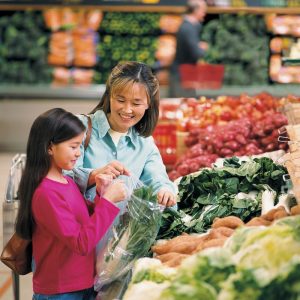
The old adage “you are what you eat” might be true on more than just a physical level: The food you choose (and even what your parents and grandparents chose) is reflected in your own personal development and risk for disease in adult life (Wells, 2003). Nutrients can reverse or change DNA methylation and histone modifications, thereby modifying the expression of critical genes associated with physiologic and pathologic processes, including embryonic development, aging, and carcinogenesis. It appears that nutrients can influence the epigenome either by directly inhibiting enzymes that catalyze DNA methylation or histone modifications, or by altering the availability of substrates necessary for those enzymatic reactions. For example, rat mothers fed a diet low in methyl group donors during pregnancy produce offspring with reduced DNMT-1 expression, decreased DNA methylation, and increased histone acetylation at promoter regions of specific genes, including the glucocorticoid receptor, and increased gene expression in the liver of juvenile offspring (Lillycrop, Phillips, Jackson, Hanson, & Burdge, 2005) and adult offspring (Lillycrop et al., 2007). These data suggest that early life nutrition has the potential to influence epigenetic programming in the brain not only during early development but also in adult life, thereby modulating health throughout life. In this regard, nutritional epigenetics has been viewed as an attractive tool to prevent pediatric developmental diseases and cancer, as well as to delay aging-associated processes.
The best evidence relating to the impact of adverse environmental conditions development and health comes from studies of the children of women who were pregnant during two civilian famines of World War II: the Siege of Leningrad (1941–44) (Bateson, 2001) and the Dutch Hunger Winter (1944–1945) (Stanner et al., 1997). In the Netherlands famine, women who were previously well nourished were subjected to low caloric intake and associated environmental stressors. Women who endured the famine in the late stages of pregnancy gave birth to smaller babies (Lumey & Stein, 1997) and these children had an increased risk of insulin resistance later in life (Painter, Roseboom, & Bleker, 2005). In addition, offspring who were starved prenatally later experienced impaired glucose tolerance in adulthood, even when food was more abundant (Stanner et al., 1997). Famine exposure at various stages of gestation was associated with a wide range of risks such as increased obesity, higher rates of coronary heart disease, and lower birth weight (Lumey & Stein, 1997). Interestingly, when examined 60 years later, people exposed to famine prenatally showed reduced DNA methylation compared with their unexposed same-sex siblings (Heijmans et al., 2008).
Epigenetic regulation of learning and memory
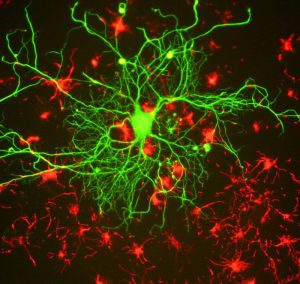
Memories are recollections of actual events stored within our brains. But how is our brain able to form and store these memories? Epigenetic mechanisms influence genomic activities in the brain to produce long-term changes in synaptic signaling, organization, and morphology, which in turn support learning and memory (Day & Sweatt, 2011).
Neuronal activity in the hippocampus of mice is associated with changes in DNA methylation (Guo et al., 2011), and disruption to genes encoding the DNA methylation machinery cause learning and memory impairments (Feng et al., 2010). DNA methylation has also been implicated in the maintenance of long-term memories, as pharmacological inhibition of DNA methylation and impaired memory (Day & Sweatt, 2011; Miller et al., 2010). These findings indicate the importance of DNA methylation in mediating synaptic plasticity and cognitive functions, both of which are disturbed in psychological illness.
Changes in histone modifications can also influence long-term memory formation by altering chromatin accessibility and the expression of genes relevant to learning and memory. Memory formation and the associated enhancements in synaptic transmission are accompanied by increases in histone acetylation (Guan et al., 2002) and alterations in histone methylation (Schaefer et al., 2009), which promote gene expression. Conversely, a neuronal increase in histone deacetylase activity, which promotes gene silencing, results in reduced synaptic plasticity and impairs memory (Guan et al., 2009). Pharmacological inhibition of histone deacetylases augments memory formation (Guan et al., 2009; Levenson et al., 2004), further suggesting that histone (de)acetylation regulates this process.
In humans genetic defects in genes encoding the DNA methylation and chromatin machinery exhibit profound effects on cognitive function and mental health (Jiang, Bressler, & Beaudet, 2004). The two best-characterized examples are Rett syndrome (Amir et al., 1999) and Rubinstein-Taybi syndrome (RTS) (Alarcon et al., 2004), which are profound intellectual disability disorders. Both MECP2 and CBP are highly expressed in neurons and are involved in regulating neural gene expression (Chen et al., 2003; Martinowich et al., 2003).
Rett syndrome patients have a mutation in their DNA sequence in a gene called MECP2. MECP2 plays many important roles within the cell: One of these roles is to read the DNA sequence, checking for DNA methylation, and to bind to areas that contain methylation, thereby preventing the wrong proteins from being present. Other roles for MECP2 include promoting the presence of particular, necessary, proteins, ensuring that DNA is packaged properly within the cell and assisting with the production of proteins. MECP2 function also influences gene expression that supports dendritic and synaptic development and hippocampus-dependent memory (Li, Zhong, Chau, Williams, & Chang, 2011; Skene et al., 2010). Mice with altered MECP2 expression exhibit genome-wide increases in histone acetylation, neuron cell death, increased anxiety, cognitive deficits, and social withdrawal (Shahbazian et al., 2002). These findings support a model in which DNA methylation and MECP2 constitute a cell-specific epigenetic mechanism for regulation of histone modification and gene expression, which may be disrupted in Rett syndrome.
RTS patients have a mutation in their DNA sequence in a gene called CBP. One of these roles of CBP is to bind to specific histones and promote histone acetylation, thereby promoting gene expression. Consistent with this function, RTS patients exhibit a genome-wide decrease in histone acetylation and cognitive dysfunction in adulthood (Kalkhoven et al., 2003). The learning and memory deficits are attributed to disrupted neural plasticity (Korzus, Rosenfeld, & Mayford, 2004). Similar to RTS in humans, mice with a mutation of CBP perform poorly in cognitive tasks and show decreased genome-wide histone acetylation (for review, see Josselyn, 2005). In the mouse brain CBP was found to act as an epigenetic switch to promote the birth of new neurons in the brain. Interestingly, this epigenetic mechanism is disrupted in the fetal brains of mice with a mutation of CBP, which, as pups, exhibit early behavioral deficits following removal and separation from their mother (Wang et al., 2010). These findings provide a novel mechanism whereby environmental cues, acting through histone modifying enzymes, can regulate epigenetic status and thereby directly promote neurogenesis, which regulates neurobehavioral development.
Together, these studies demonstrate that misregulation of epigenetic modifications and their regulatory enzymes is capable of orchestrating prominent deficits in neuronal plasticity and cognitive function. Knowledge from these studies may provide greater insight into other mental disorders such as depression and suicidal behaviors.
Epigenetic mechanisms in psychological disorders
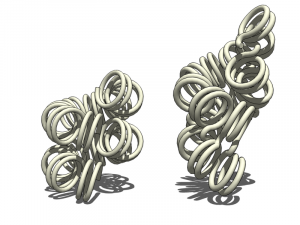
Epigenome-wide studies have identified several dozen sites with DNA methylation alterations in genes involved in brain development and neurotransmitter pathways, which had previously been associated with mental illness (Mill et al., 2008). These disorders are complex and typically start at a young age and cause lifelong disability. Often, limited benefits from treatment make these diseases some of the most burdensome disorders for individuals, families, and society. It has become evident that the efforts to identify the primary causes of complex psychiatric disorders may significantly benefit from studies linking environmental effects with changes observed within the individual cells.
Epigenetic events that alter chromatin structure to regulate programs of gene expression have been associated with depression-related behavior and action of antidepressant medications, with increasing evidence for similar mechanisms occurring in post-mortem brains of depressed individuals. In mice, social avoidance resulted in decreased expression of hippocampal genes important in mediating depressive responses (Tsankova et al., 2006). Similarly, chronic social defeat stress was found to decrease expression of genes implicated in normal emotion processing (Lutter et al., 2008). Consistent with these findings, levels of histone markers of increased gene expression were down regulated in human post-mortem brain samples from individuals with a history of clinical depression (Covington et al., 2009).
Administration of antidepressants increased histone markers of increased gene expression and reversed the gene repression induced by defeat stress (Lee, Wynder, Schmidt, McCafferty, & Shiekhattar, 2006; Tsankova et al., 2006; Wilkinson et al., 2009). These results provide support for the use of HDAC inhibitors against depression. Accordingly, several HDAC inhibitors have been found to exert antidepressant effects by each modifying distinct cellular targets (Cassel et al., 2006; Schroeder, Lin, Crusio, & Akbarian, 2007).
There is also increasing evidence that aberrant gene expression resulting from altered epigenetic regulation is associated with the pathophysiology of suicide (McGowan et al., 2008; Poulter et al., 2008). Thus, it is tempting to speculate that there is an epigenetically determined reduced capacity for gene expression, which is required for learning and memory, in the brains of suicide victims.
Epigenetic strategy to understanding gene-environment interactions
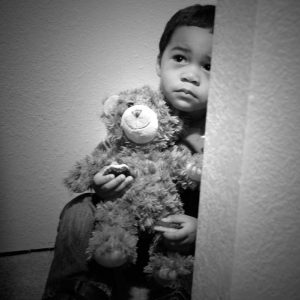
While the cellular and molecular mechanisms that influence on physical and mental health have long been a central focus of neuroscience, only in recent years has attention turned to the epigenetic mechanisms behind the dynamic changes in gene expression responsible for normal cognitive function and increased risk for mental illness. The links between early environment and epigenetic modifications suggest a mechanism underlying gene-environment interactions. Early environmental adversity alone is not a sufficient cause of mental illness, because many individuals with a history of severe childhood maltreatment or trauma remain healthy. It is increasingly becoming evident that inherited differences in the segments of specific genes may moderate the effects of adversity and determine who is sensitive and who is resilient through a gene-environment interplay. Genes such as the glucocorticoid receptor appear to moderate the effects of childhood adversity on mental illness. Remarkably, epigenetic DNA modifications have been identified that may underlie the long-lasting effects of environment on biological functions. This new epigenetic research is pointing to a new strategy to understanding gene-environment interactions.
The next decade of research will show if this potential can be exploited in the development of new therapeutic options that may alter the traces that early environment leaves on the genome. However, as discussed in this module, the epigenome is not static and can be molded by developmental signals, environmental perturbations, and disease states, which present an experimental challenge in the search for epigenetic risk factors in psychological disorders (Rakyan, Down, Balding, & Beck, 2011). The sample size and epigenomic assay required is dependent on the number of tissues affected, as well as the type and distribution of epigenetic modifications. The combination of genetic association maps studies with epigenome-wide developmental studies may help identify novel molecular mechanisms to explain features of inheritance of personality traits and transform our understanding of the biological basis of psychology. Importantly, these epigenetic studies may lead to identification of novel therapeutic targets and enable the development of improved strategies for early diagnosis, prevention, and better treatment of psychological and behavioral disorders.
A "Big Picture" Insight into Epi-Genetics
Epigentics is a content area where students sometimes feel apprehensive, especially if they do not have a background in biology. This course will teach you all required biology content expected for this class. To help get you excited about the importance of this research field, please watch the following video. It provides insights into why it is so important for behavioural scientists to understand, at least on a surface level, about the field of epigenetics. After you watch it, please be sure to go through this module again. This video is not intended as a replacement for the content in this module, but rather is intended to serve as a big-picture framework for you to better understand the content in this module.
Check Your Knowledge
To help you with your studying, we’ve included some practice questions for this module. These questions do not necessarily address all content in this module. They are intended as practice, and you are responsible for all of the content in this module even if there is no associated practice question. To promote deeper engagement with the material, we encourage you to create some questions of your own for your practice. You can then also return to these self-generated questions later in the course to test yourself.
Vocabulary
- DNA methylation
- Covalent modifications of mammalian DNA occurring via the methylation of cytosine, typically in the context of the CpG dinucleotide.
- DNA methyltransferases (DNMTs)
- Enzymes that establish and maintain DNA methylation using methyl-group donor compounds or cofactors. The main mammalian DNMTs are DNMT1, which maintains methylation state across DNA replication, and DNMT3a and DNMT3b, which perform de novo methylation.
- Epigenetics
- The study of heritable changes in gene expression or cellular phenotype caused by mechanisms other than changes in the underlying DNA sequence. Epigenetic marks include covalent DNA modifications and posttranslational histone modifications.
- Epigenome
- The genome-wide distribution of epigenetic marks.
- Gene
- A specific deoxyribonucleic acid (DNA) sequence that codes for a specific polypeptide or protein or an observable inherited trait.
- Genome-wide association study (GWAS)
- A study that maps DNA polymorphisms in affected individuals and controls matched for age, sex, and ethnic background with the aim of identifying causal genetic variants.
- Genotype
- The DNA content of a cell’s nucleus, whether a trait is externally observable or not.
- Histone acetyltransferases (HATs) and histone deacetylases (HDACs)
- HATs are enzymes that transfer acetyl groups to specific positions on histone tails, promoting an “open” chromatin state and transcriptional activation. HDACs remove these acetyl groups, resulting in a “closed” chromatin state and transcriptional repression.
- Histone modifications
- Posttranslational modifications of the N-terminal “tails” of histone proteins that serve as a major mode of epigenetic regulation. These modifications include acetylation, phosphorylation, methylation, sumoylation, ubiquitination, and ADP-ribosylation.
- Identical twins
- Two individual organisms that originated from the same zygote and therefore are genetically identical or very similar. The epigenetic profiling of identical twins discordant for disease is a unique experimental design as it eliminates the DNA sequence-, age-, and sex-differences from consideration.
- Phenotype
- The pattern of expression of the genotype or the magnitude or extent to which it is observably expressed—an observable characteristic or trait of an organism, such as its morphology, development, biochemical or physiological properties, or behavior.
References
- Adams, R. L., McKay, E. L., Craig, L. M., & Burdon, R. H. (1979). Mouse DNA methylase: methylation of native DNA. Biochimica et Biophysica Acta, 561(2), 345–357.
- Alarcon, J. M., Malleret, G., Touzani, K., Vronskaya, S., Ishii, S., Kandel, E. R., & Barco, A. (2004). Chromatin acetylation, memory, and LTP are impaired in CBP+/- mice: a model for the cognitive deficit in Rubinstein-Taybi syndrome and its amelioration. Neuron, 42(6), 947–959. doi: 10.1016/j.neuron.2004.05.021, S0896627304003022 [pii]
- Amir, R. E., Van den Veyver, I. B., Wan, M., Tran, C. Q., Francke, U., & Zoghbi, H. Y. (1999). Rett syndrome is caused by mutations in X-linked MECP2, encoding methyl-CpG-binding protein 2. Nature Genetics, 23(2), 185–188.
- Bateson, P. (2001). Fetal experience and good adult design. International Journal of Epidemiology, 30(5), 928–934.
- Bradshaw, A.D. (1965). Evolutionary significance of phenotypic plasticity in plants. Advances in Genetics, 13, 115–155.
- Caldji, C., Tannenbaum, B., Sharma, S., Francis, D., Plotsky, P. M., & Meaney, M. J. (1998). Maternal care during infancy regulates the development of neural systems mediating the expression of fearfulness in the rat. Proceedings of the National Academy of Sciences U S A, 95(9), 5335–5340.
- Cassel, S., Carouge, D., Gensburger, C., Anglard, P., Burgun, C., Dietrich, J. B., . . . Zwiller, J. (2006). Fluoxetine and cocaine induce the epigenetic factors MeCP2 and MBD1 in adult rat brain. Molecular Pharmacology, 70(2), 487–492. doi: 10.1124/mol.106.022301
- Chen, W. G., Chang, Q., Lin, Y., Meissner, A., West, A. E., Griffith, E. C., . . . Greenberg, M. E. (2003). Derepression of BDNF transcription involves calcium-dependent phosphorylation of MeCP2. Science, 302(5646), 885–889.
- Covington, H. E., 3rd, Maze, I., LaPlant, Q. C., Vialou, V. F., Ohnishi, Y. N., Berton, O., . . . Nestler, E. J. (2009). Antidepressant actions of histone deacetylase inhibitors. Journal of Neuroscience, 29(37), 11451–11460. doi: 10.1523/JNEUROSCI.1758-09.2009
- Davie, J. R., & Chadee, D. N. (1998). Regulation and regulatory parameters of histone modifications. *Journal of Cellular Biochemistry Suppl, 30–31 *, 203–213.
- Day, J. J., & Sweatt, J. D. (2011). Epigenetic mechanisms in cognition. Neuron, 70(5), 813–829. doi: 10.1016/j.neuron.2011.05.019
- Dick, D. M., Riley, B., & Kendler, K. S. (2010). Nature and nurture in neuropsychiatric genetics: where do we stand? Dialogues in Clinical Neuroscience, 12(1), 7–23.
- Dolinoy, D. C., Weidman, J. R., & Jirtle, R. L. (2007). Epigenetic gene regulation: linking early developmental environment to adult disease. Reproductive Toxicology, 23(3), 297–307. doi: S0890-6238(06)00197-3 [pii], 10.1016/j.reprotox.2006.08.012
- Feng, J., Chang, H., Li, E., & Fan, G. (2005). Dynamic expression of de novo DNA methyltransferases Dnmt3a and Dnmt3b in the central nervous system. Journal of Neuroscience Research, 79(6), 734–746. doi: 10.1002/jnr.20404
- Feng, J., Zhou, Y., Campbell, S. L., Le, T., Li, E., Sweatt, J. D., . . . Fan, G. (2010). Dnmt1 and Dnmt3a maintain DNA methylation and regulate synaptic function in adult forebrain neurons. Nature Neuroscience, 13(4), 423–430. doi: 10.1038/nn.2514
- Francis, D., Diorio, J., Liu, D., & Meaney, M. J. (1999). Nongenomic transmission across generations of maternal behavior and stress responses in the rat. Science, 286(5442), 1155–1158.
- Gershon, E. S., Alliey-Rodriguez, N., & Liu, C. (2011). After GWAS: searching for genetic risk for schizophrenia and bipolar disorder. American Journal of Psychiatry, 168(3), 253–256. doi: 10.1176/appi.ajp.2010.10091340
- Goll, M. G., & Bestor, T. H. (2005). Eukaryotic cytosine methyltransferases. Annual Review of Biochemistry, 74, 481–514. doi: 10.1146/annurev.biochem.74.010904.153721
- Goto, K., Numata, M., Komura, J. I., Ono, T., Bestor, T. H., & Kondo, H. (1994). Expression of DNA methyltransferase gene in mature and immature neurons as well as proliferating cells in mice. Differentiation, 56(1–2), 39–44.
- Gregg, C., Zhang, J., Weissbourd, B., Luo, S., Schroth, G. P., Haig, D., & Dulac, C. (2010). High-resolution analysis of parent-of-origin allelic expression in the mouse brain. Science, 329(5992), 643–648. doi: 10.1126/science.1190830
- Guan, J. S., Haggarty, S. J., Giacometti, E., Dannenberg, J. H., Joseph, N., Gao, J., . . . Tsai, L. H. (2009). HDAC2 negatively regulates memory formation and synaptic plasticity. Nature, 459(7243), 55-60. doi: 10.1038/nature07925
- Guan, Z., Giustetto, M., Lomvardas, S., Kim, J. H., Miniaci, M. C., Schwartz, J. H., . . . Kandel, E. R. (2002). Integration of long-term-memory-related synaptic plasticity involves bidirectional regulation of gene expression and chromatin structure. Cell, 111(4), 483–493.
- Guo, J. U., Ma, D. K., Mo, H., Ball, M. P., Jang, M. H., Bonaguidi, M. A., . . . Song, H. (2011). Neuronal activity modifies the DNA methylation landscape in the adult brain. Nature Neuroscience, 14(10), 1345–1351. doi: 10.1038/nn.2900
- Heijmans, B. T., Tobi, E. W., Stein, A. D., Putter, H., Blauw, G. J., Susser, E. S., . . . Lumey, L. H. (2008). Persistent epigenetic differences associated with prenatal exposure to famine in humans. Proceedings of the National Academy of Sciences U S A, 105(44), 17046–17049. doi: 0806560105 [pii]10.1073/pnas.0806560105
- Hong, L., Schroth, G. P., Matthews, H. R., Yau, P., & Bradbury, E. M. (1993). Studies of the DNA binding properties of histone H4 amino terminus. Thermal denaturation studies reveal that acetylation markedly reduces the binding constant of the H4 "tail" to DNA. Journal of Biological Chemistry, 268(1), 305–314.
- Jenuwein, T., & Allis, C. D. (2001). Translating the histone code. Science, 293(5532), 1074–1080. doi: 10.1126/Science.1063127293/5532/1074 [pii]
- Jiang, Y. H., Bressler, J., & Beaudet, A. L. (2004). Epigenetics and human disease. Annual Review of Genomics and Human Genetics, 5, 479–510. doi: 10.1146/annurev.genom.5.061903.180014
- Josselyn, S. A. (2005). What's right with my mouse model? New insights into the molecular and cellular basis of cognition from mouse models of Rubinstein-Taybi Syndrome. Learning & Memory, 12(2), 80–83. doi: 12/2/80 [pii]10.1101/lm.93505
- Kadonaga, J. T. (1998). Eukaryotic transcription: an interlaced network of transcription factors and chromatin-modifying machines. Cell, 92(3), 307–313.
- Kalkhoven, E., Roelfsema, J. H., Teunissen, H., den Boer, A., Ariyurek, Y., Zantema, A., . . . Peters, D. J. (2003). Loss of CBP acetyltransferase activity by PHD finger mutations in Rubinstein-Taybi syndrome. Human Molecular Genetics, 12(4), 441–450.
- Korzus, E., Rosenfeld, M. G., & Mayford, M. (2004). CBP histone acetyltransferase activity is a critical component of memory consolidation. Neuron, 42(6), 961–972. doi: 10.1016/j.neuron.2004.06.002S0896627304003526 [pii]
- Kuo, M. H., & Allis, C. D. (1998). Roles of histone acetyltransferases and deacetylases in gene regulation. Bioessays, 20(8), 615–626. doi: 10.1002/(SICI)1521–1878(199808)20:8<615::AID-BIES4>3.0.CO;2-H [pii] 10.1002/(SICI)1521-1878(199808)20:8<615::AID-BIES4>3.0.CO;2-H
- Law, J. A., & Jacobsen, S. E. (2010). Establishing, maintaining and modifying DNA methylation patterns in plants and animals. Nature Reviews Genetics, 11(3), 204–220. doi: nrg2719 [pii]10.1038/nrg2719
- Lee, M. G., Wynder, C., Schmidt, D. M., McCafferty, D. G., & Shiekhattar, R. (2006). Histone H3 lysine 4 demethylation is a target of nonselective antidepressive medications. Chemistry & Biology, 13(6), 563–567. doi: 10.1016/j.chembiol.2006.05.004
- Levenson, J. M., O'Riordan, K. J., Brown, K. D., Trinh, M. A., Molfese, D. L., & Sweatt, J. D. (2004). Regulation of histone acetylation during memory formation in the hippocampus. Journal of Biological Chemistry, 279(39), 40545–40559.
- Li, H., Zhong, X., Chau, K. F., Williams, E. C., & Chang, Q. (2011). Loss of activity-induced phosphorylation of MeCP2 enhances synaptogenesis, LTP and spatial memory. Nature Neuroscience, 14(8), 1001–1008. doi: 10.1038/nn.2866
- Lillycrop, K. A., Phillips, E. S., Jackson, A. A., Hanson, M. A., & Burdge, G. C. (2005). Dietary protein restriction of pregnant rats induces and folic acid supplementation prevents epigenetic modification of hepatic gene expression in the offspring. Journal of Nutrition, 135(6), 1382–1386. doi: 135/6/1382 [pii]
- Lillycrop, K. A., Slater-Jefferies, J. L., Hanson, M. A., Godfrey, K. M., Jackson, A. A., & Burdge, G. C. (2007). Induction of altered epigenetic regulation of the hepatic glucocorticoid receptor in the offspring of rats fed a protein-restricted diet during pregnancy suggests that reduced DNA methyltransferase-1 expression is involved in impaired DNA methylation and changes in histone modifications. British Journal of Nutrition, 97(6), 1064–1073. doi: S000711450769196X [pii]10.1017/S000711450769196X
- Liu, D., Diorio, J., Tannenbaum, B., Caldji, C., Francis, D., Freedman, A., . . . Meaney, M. J. (1997). Maternal care, hippocampal glucocorticoid receptors, and hypothalamic- pituitary-adrenal responses to stress [see comments]. Science, 277(5332), 1659–1662.
- Lumey, L. H., & Stein, A. D. (1997). Offspring birth weights after maternal intrauterine undernutrition: a comparison within sibships. American Journal of Epidemiology, 146(10), 810–819.
- Lutter, M., Krishnan, V., Russo, S. J., Jung, S., McClung, C. A., & Nestler, E. J. (2008). Orexin signaling mediates the antidepressant-like effect of calorie restriction. Journal of Neuroscience, 28(12), 3071–3075. doi: 10.1523/JNEUROSCI.5584-07.2008
- Martinowich, K., Hattori, D., Wu, H., Fouse, S., He, F., Hu, Y., . . . Sun, Y. E. (2003). DNA methylation-related chromatin remodeling in activity-dependent BDNF gene regulation. Science, 302(5646), 890–893.
- McGowan, P. O., Sasaki, A., D'Alessio, A. C., Dymov, S., Labonte, B., Szyf, M., . . . Meaney, M. J. (2009). Epigenetic regulation of the glucocorticoid receptor in human brain associates with childhood abuse. Nature Neuroscience, 12(3), 342–348. doi: nn.2270 [pii]10.1038/nn.2270
- McGowan, P. O., Sasaki, A., Huang, T. C., Unterberger, A., Suderman, M., Ernst, C., . . . Szyf, M. (2008). Promoter-wide hypermethylation of the ribosomal RNA gene promoter in the suicide brain. PLoS ONE, 3(5), e2085. doi: 10.1371/journal.pone.0002085
- Mill, J., Tang, T., Kaminsky, Z., Khare, T., Yazdanpanah, S., Bouchard, L., . . . Petronis, A. (2008). Epigenomic profiling reveals DNA-methylation changes associated with major psychosis. American Journal of Human Genetics, 82(3), 696–711. doi: 10.1016/j.ajhg.2008.01.008
- Miller, C. A., Gavin, C. F., White, J. A., Parrish, R. R., Honasoge, A., Yancey, C. R., . . . Sweatt, J. D. (2010). Cortical DNA methylation maintains remote memory. Nature Neuroscience, 13(6), 664–666. doi: 10.1038/nn.2560
- Myers, M. M., Brunelli, S. A., Shair, H. N., Squire, J. M., & Hofer, M. A. (1989). Relationships between maternal behavior of SHR and WKY dams and adult blood pressures of cross-fostered F1 pups. Developmental Psychobiology, 22(1), 55–67.
- Oberlander, T. F., Weinberg, J., Papsdorf, M., Grunau, R., Misri, S., & Devlin, A. M. (2008). Prenatal exposure to maternal depression, neonatal methylation of human glucocorticoid receptor gene (NR3C1) and infant cortisol stress responses. Epigenetics, 3(2), 97–106. doi: 6034 [pii]
- Ooi, S. K., O'Donnell, A. H., & Bestor, T. H. (2009). Mammalian cytosine methylation at a glance. Journal of Cell Science, 122(Pt 16), 2787–2791. doi: 122/16/2787 [pii]10.1242/jcs.015123
- Painter, R. C., Roseboom, T. J., & Bleker, O. P. (2005). Prenatal exposure to the Dutch famine and disease in later life: an overview. Reproductive Toxicology, 20(3), 345–352. doi: S0890-6238(05)00088-2 [pii]10.1016/j. Reproductive Toxicology.2005.04.005
- Petronis, A. (2010). Epigenetics as a unifying principle in the aetiology of complex traits and diseases. Nature, 465(7299), 721–727. doi: 10.1038/nature09230
- Poulter, M. O., Du, L., Weaver, I. C., Palkovits, M., Faludi, G., Merali, Z., . . . Anisman, H. (2008). GABAA receptor promoter hypermethylation in suicide brain: implications for the involvement of epigenetic processes. Biological Psychiatry, 64(8), 645–652. doi: 10.1016/j.biopsych.2008.05.028
- Rakyan, V. K., Down, T. A., Balding, D. J., & Beck, S. (2011). Epigenome-wide association studies for common human diseases. Nature Reviews Genetics, 12(8), 529–541. doi: 10.1038/nrg3000
- Razin, A. (1998). CpG methylation, chromatin structure and gene silencing-a three-way connection. European Molecular Biology Organization, 17(17), 4905–4908.
- Schaefer, A., Sampath, S. C., Intrator, A., Min, A., Gertler, T. S., Surmeier, D. J., . . . Greengard, P. (2009). Control of cognition and adaptive behavior by the GLP/G9a epigenetic suppressor complex. Neuron, 64(5), 678–691. doi: 10.1016/j.neuron.2009.11.019
- Schroeder, F. A., Lin, C. L., Crusio, W. E., & Akbarian, S. (2007). Antidepressant-like effects of the histone deacetylase inhibitor, sodium butyrate, in the mouse. Biological Psychiatry, 62(1), 55-64. doi: 10.1016/j.biopsych.2006.06.036
- Sealy, L., & Chalkley, R. (1978). DNA associated with hyperacetylated histone is preferentially digested by DNase I. Nucleic Acids Research, 5(6), 1863–1876.
- Shahbazian, M., Young, J., Yuva-Paylor, L., Spencer, C., Antalffy, B., Noebels, J., . . . Zoghbi, H. (2002). Mice with truncated MeCP2 recapitulate many Rett syndrome features and display hyperacetylation of histone H3. Neuron, 35(2), 243–254.
- Skene, P. J., Illingworth, R. S., Webb, S., Kerr, A. R., James, K. D., Turner, D. J., . . . Bird, A. P. (2010). Neuronal MeCP2 is expressed at near histone-octamer levels and globally alters the chromatin state. Molecular Cell, 37(4), 457–468. doi: 10.1016/j.molcel.2010.01.030
- Stanner, S. A., Bulmer, K., Andres, C., Lantseva, O. E., Borodina, V., Poteen, V. V., & Yudkin, J. S. (1997). Does malnutrition in utero determine diabetes and coronary heart disease in adulthood? Results from the Leningrad siege study, a cross sectional study. British Medical Journal, 315(7119), 1342–1348.
- Stern, J. M. (1997). Offspring-induced nurturance: animal-human parallels. Developmental Psychobiololgy, 31(1), 19–37.
- Sutter, D., Doerfler, W., 1980. Methylation of integrated adenovirus type 12 DNA sequences in transformed cells is inversely correlated with viral gene expression. Proceedings of the National Academy of Sciences U S A. 77, 253–256.
- Suzuki, M. M., & Bird, A. (2008). DNA methylation landscapes: provocative insights from epigenomics. Nature Reviews Genetics, 9(6), 465–476. doi: nrg2341 [pii]10.1038/nrg2341
- Tsankova, N. M., Berton, O., Renthal, W., Kumar, A., Neve, R. L., & Nestler, E. J. (2006). Sustained hippocampal chromatin regulation in a mouse model of depression and antidepressant action. Nature Neuroscience. 9(4): 519–525. doi:10.1038/nn1659
- Turner, J. D., Pelascini, L. P., Macedo, J. A., & Muller, C. P. (2008). Highly individual methylation patterns of alternative glucocorticoid receptor promoters suggest individualized epigenetic regulatory mechanisms. Nucleic Acids Research, 36(22), 7207–7218. doi: gkn897 [pii] 10.1093/nar/gkn897
- Vardimon, L., Kressmann, A., Cedar, H., Maechler, M., Doerfler, W., 1982. Expression of a cloned adenovirus gene is inhibited by in vitro methylation. Proceedings of the National Academy of Sciences U S A. 79, 1073–1077.
- Waddington, C. H. (1942). Epigenotype. Endeavour(1), 18–21.
- Wade, P. A., Pruss, D., & Wolffe, A. P. (1997). Histone acetylation: chromatin in action. Trends in Biochemical Sciences, 22(4), 128–132. doi: S0968000497010165 [pii]
- Wang, J., Weaver, I. C., Gauthier-Fisher, A., Wang, H., He, L., Yeomans, J., . . . Miller, F. D. (2010). CBP histone acetyltransferase activity regulates embryonic neural differentiation in the normal and Rubinstein-Taybi syndrome brain. Developmental Cell, 18(1), 114–125. doi: 10.1016/j.devcel.2009.10.023
- Weaver, I. C., Cervoni, N., Champagne, F. A., D'Alessio, A. C., Sharma, S., Seckl, J. R., . . . Meaney, M. J. (2004). Epigenetic programming by maternal behavior. Nature Neuroscience, 7(8), 847–854. doi: 10.1038/nn1276
- Weaver, I. C., Champagne, F. A., Brown, S. E., Dymov, S., Sharma, S., Meaney, M. J., & Szyf, M. (2005). Reversal of maternal programming of stress responses in adult offspring through methyl supplementation: altering epigenetic marking later in life. Journal of Neuroscience, 25(47), 11045–11054. doi: 10.1523/JNEUROSCI.3652-05.2005
- Weaver, I. C., Meaney, M. J., & Szyf, M. (2006). Maternal care effects on the hippocampal transcriptome and anxiety-mediated behaviors in the offspring that are reversible in adulthood. Proceedings of the National Academy of Sciences U S A, 103(9), 3480–3485. doi: 10.1073/pnas.0507526103
- Wells, J. C. (2003). The thrifty phenotype hypothesis: thrifty offspring or thrifty mother? Journal of Theoretical Biology, 221(1), 143–161.
- Wilkinson, M. B., Xiao, G., Kumar, A., LaPlant, Q., Renthal, W., Sikder, D., . . . Nestler, E. J. (2009). Imipramine treatment and resiliency exhibit similar chromatin regulation in the mouse nucleus accumbens in depression models. Journal of Neuroscience, 29(24), 7820–7832. doi: 10.1523/JNEUROSCI.0932-09.2009
- Wolffe, A. P., & Matzke, M. A. (1999). Epigenetics: regulation through repression. Science, 286(5439), 481–486.
How to cite this Chapter using APA Style:
Weaver, I. (2019). Epigenetics in psychology. Adapted for use by Queen's University. Original chapter in R. Biswas-Diener & E. Diener (Eds), Noba textbook series: Psychology.Champaign, IL: DEF publishers. Retrieved from http://noba.to/37p5cb8v
Copyright and Acknowledgment:
This material is licensed under the Creative Commons Attribution-NonCommercial-ShareAlike 4.0 International License. To view a copy of this license, visit: http://creativecommons.org/licenses/by-nc-sa/4.0/deed.en_US.
This material is attributed to the Diener Education Fund (copyright © 2018) and can be accessed via this link: http://noba.to/37p5cb8v.
Additional information about the Diener Education Fund (DEF) can be accessed here.
Original chapter by M. Brent Donnellan adapted by the Queen’s University Psychology Department
This Open Access chapter was originally written for the NOBA project. Information on the NOBA project can be found below.
This module describes different ways to address questions about personality stability across the lifespan. Definitions of the major types of personality stability are provided, and evidence concerning the different kinds of stability and change are reviewed. The mechanisms thought to produce personality stability and personality change are identified and explained.
Learning Objectives
- Define heterotypic stability, homotypic stability, absolute stability, and differential stability.
- Describe evidence concerning the absolute and differential stability of personality attributes across the lifespan.
- Explain the maturity, cumulative continuity, and corresponsive principles of personality development.
- Explain person-environment transactions, and distinguish between active, reactive, and evocative person-environment transactions.
- Identify the four processes that promote personality stability (attraction, selection, manipulation, and attrition). Provide examples of these processes.
- Describe the mechanisms behind the possibility of personality transformation.
Introduction
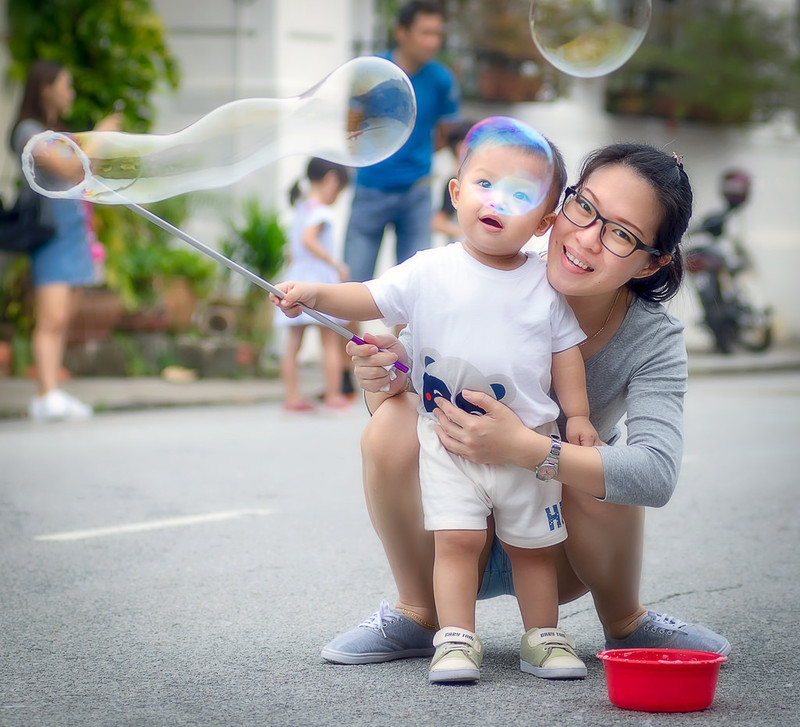
Personality psychology is about how individuals differ from each other in their characteristic ways of thinking, feeling, and behaving. Some of the most interesting questions about personality attributes involve issues of stability and change. Are shy children destined to become shy adults? Are the typical personality attributes of adults different from the typical attributes of adolescents? Do people become more self-controlled and better able to manage their negative emotions as they become adults? What mechanisms explain personality stability and what mechanisms account for personality change?
Defining Different Kinds of Personality Stability
Something frustrating happens when you attempt to learn about personality stability[1]: As with many topics in psychology, there are a number of different ways to conceptualize and quantify personality stability (e.g., Caspi & Bem, 1990; Roberts, Wood, & Caspi, 2008). This means there are multiple ways to consider questions about personality stability. Thus, the simple (and obviously frustrating) way to respond to most blanket questions about personality stability is to simply answer that it depends on what one means by personality stability. To provide a more satisfying answer to questions about stability, I will first describe the different ways psychologists conceptualize and evaluate personality stability. I will make an important distinction between heterotypic and homotypic stability. I will then describe absolute and differential stability, two ways of considering homotypic stability. I will also draw your attention to the important concept of individual differences in personality development.
Heterotypic stability refers to the psychological coherence of an individual’s thoughts, feelings, and behaviors across development. Questions about heterotypic stability concern the degree of consistency in underlying personality attributes. The tricky part of studying heterotypic stability is that the underlying psychological attribute can have different behavioral expressions at different ages. (You may already know that the prefix “hetero” means something like “different” in Greek.) Shyness is a good example of such an attribute because shyness is expressed differently by toddlers and young children than adults. The shy toddler might cling to a caregiver in a crowded setting and burst into tears when separated from this caregiver. The shy adult, on the other hand, may avoid making eye contact with strangers and seem aloof and distant at social gatherings. It would be highly unusual to observe an adult burst into tears in a crowded setting. The observable behaviors typically associated with shyness “look” different at different ages. Researchers can study heterotypic continuity only once they have a theory that specifies the different behavioral manifestations of the psychological attribute at different points in the lifespan. As it stands, there is evidence that attributes such as shyness and aggression exhibit heterotypic stability across the lifespan (Caspi, Bem, & Elder, 1989). Individuals who act shy as children often act shy as adults, but the degree of correspondence is far from perfect because many things can intervene between childhood and adulthood to alter how an individual develops. Nonetheless, the important point is that the patterns of behavior observed in childhood sometimes foreshadow adult personality attributes.
Homotypic stability concerns the amount of similarity in the same observable personality characteristics across time. (The prefix “homo” means something like the “same” in Greek.) For example, researchers might ask whether stress reaction or the tendency to become easily distressed by the normal challenges of life exhibits homotypic stability from age 25 to age 45. The assumption is that this attribute has the same manifestations at these different ages. Researchers make further distinctions between absolute stability and differential stability when considering homotypic stability.
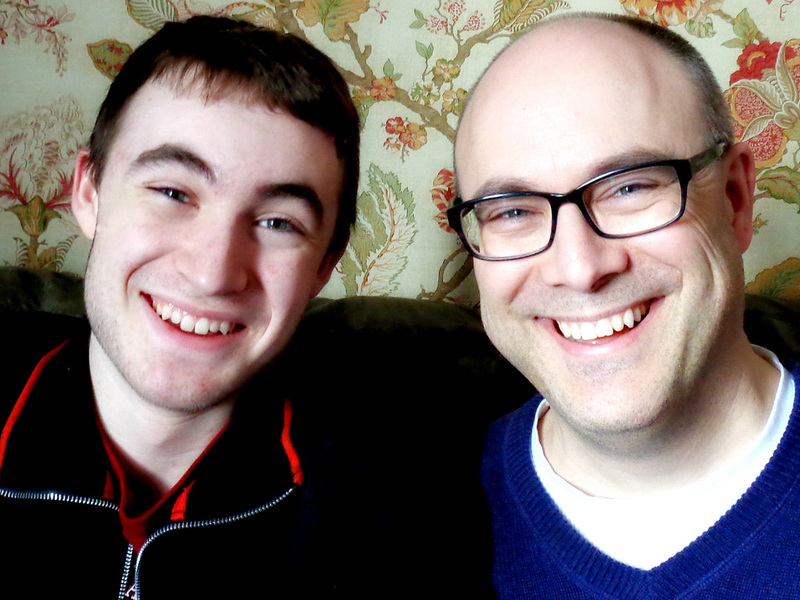
Absolute stability refers to the consistency of the level of the same personality attribute across time. If an individual received a score of 45 on a hypothetical measure of stress reaction at age 20 and at age 40, researchers would conclude there was evidence of absolute stability. Questions about absolute stability can be considered at the group level or the individual level. At the group level, it is common for personality researchers to compare average scores on personality measures for groups of different ages. For example, it is possible to investigate whether the average 40-year-old adult has a lower (or higher) level of stress reaction than the average 20-year-old. The answer to this question would tell researchers something about typical patterns of personality development.
It is important to consider absolute stability from both the group and individual perspectives. The individual level is interesting because different people might have different patterns of absolute change over time. One person might report consistently low levels of stress reaction throughout adulthood, whereas another person may report dramatic increases in stress reaction during her 30s and 40s. These different individual patterns can be present even if the overall trend is for a decline in stress reaction with age. Personality psychology is about individual differences and whether an individual’s attributes change or remain the same across time might be an important individual difference. Indeed, there are intriguing hints that the rate and direction of change in characteristics such as stress reaction (or neuroticism) predicts mortality (Mroczek & Spiro, 2007).
Differential stability refers to the consistency of a personality attribute in terms of an individual’s rank-ordering. A typical question about differential stability might be whether a 20-year-old who is low in stress reaction relative to her same aged peers develops into a 40-year-old who is also low in stress reaction compared to her peers. Differential stability is often interesting because many psychological attributes show average changes across the lifespan. Regardless of average changes with age, however, it is common to assume that more trait-like attributes have a high degree of differential stability. Consider athletic performance as an attribute that may exhibit differential stability. The average 35-year-old is likely to run a 5K race faster than the average 55-year-old. Nonetheless, individuals who are fast relative to their peers in their 30s might also be fast relative to their peers in their 50s. Likewise, even if most people decline on a stress reaction as they age, it is still useful to investigate whether there is consistency over time in their relative standing on this attribute.
Basic Findings about Absolute and Differential Stability
Absolute Stability. There are two common ways to investigate average levels of personality attributes at different ages. The simplest approach is to conduct a cross-sectional study and compare different age groups on a given attribute assessed at the same time. For instance, researchers might collect data from a sample of individuals ranging in age from 18 to 99 years and compare stress reaction scores for groups of different ages. A more complicated design involves following the same group of individuals and assessing their personalities at multiple time points (often two). This is a longitudinal study,and it is a much better way to study personality stability than a cross-sectional study. If all of the individuals in the sample are roughly the same age at the start of the study, they would all be considered members of the same birth cohort. One of the chief drawbacks of a cross-sectional study is that individuals who are of different ages are also members of different birth cohorts. Thus, researchers have no way of knowing whether any personality differences observed in a cross-sectional study are attributable to the influence of age per se or birth cohort. A longitudinal study is better able to isolate age effects (i.e., differences in personality related to maturation and development) from cohort effects (i.e., differences in personality related to being born at a particular point in history) than a cross-sectional study. Cohort is a constant (i.e., an unchanging value) in a longitudinal study when all participants start the study at roughly the same age.
A number of large-scale, cross-sectional studies have evaluated age differences in personality (Anusic, Lucas, & Donnellan, 2012; Lucas & Donnellan, 2009; McCrae & Costa, 2003; Soto, John, Gosling, & Potter, 2011; Srivastava, John, Gosling, & Potter, 2003) as have a number of longitudinal studies (Lucas & Donnellan, 2011; Specht, Egloff, & Schmukle, 2011; Terracciano, McCrae, Brant, & Costa, 2005; Wortman, Lucas, & Donnellan, in press). Fortunately, many of the general trends from these different designs converge on the same basic set of findings. Most notably, Roberts, Walton, and Viechtbauer (2006) combined the results of 92 longitudinal studies to provide an overview of absolute changes in personality across the lifespan. They used the Big Five taxonomy (e.g., John, Naumann, & Soto, 2008) to categorize the different personality attributes examined in the individual studies to make sense of the vast literature.
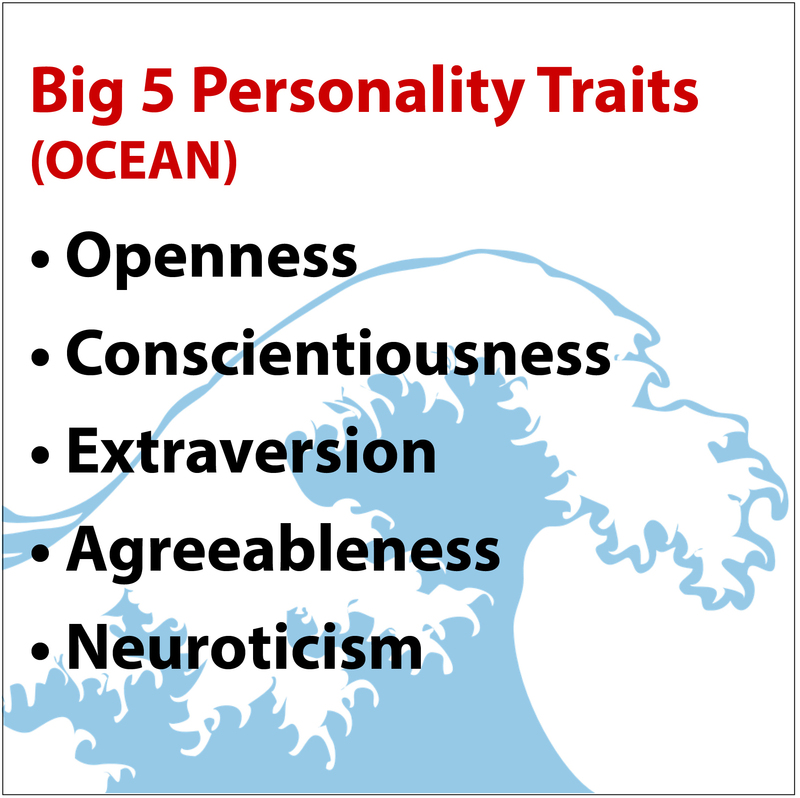
The Big Five domains include extraversion (attributes such as assertive, confident, independent, outgoing, and sociable), agreeableness (attributes such as cooperative, kind, modest, and trusting), conscientiousness (attributes such as hard working, dutiful, self-controlled, and goal-oriented), neuroticism (attributes such as anxious, tense, moody, and easily angered), and openness (attributes such as artistic, curious, inventive, and open-minded). The Big Five is one of the most common ways of organizing the vast range of personality attributes that seem to distinguish one person from the next. This organizing framework made it possible for Roberts et al. (2006) to draw broad conclusions from the literature.
In general, average levels of extraversion (especially the attributes linked to self-confidence and independence), agreeableness, and conscientiousness appear to increase with age whereas neuroticism appears to decrease with age (Roberts et al., 2006). Openness also declines with age, especially after mid-life (Roberts et al., 2006). These changes are often viewed as positive trends given that higher levels of agreeableness and conscientiousness and lower levels of neuroticism are associated with seemingly desirable outcomes such as increased relationship stability and quality, greater success at work, better health, a reduced risk of criminality and mental health problems, and even decreased mortality (e.g., Kotov, Gamez, Schmidt, & Watson, 2010; Miller & Lynam 2001; Ozer & Benet-Martínez, 2006; Roberts, Kuncel, Shiner, Caspi, & Goldberg, 2007). This pattern of positive average changes in personality attributes is known as the maturity principle of adult personality development (Caspi, Roberts, & Shiner, 2005). The basic idea is that attributes associated with positive adaptation and attributes associated with the successful fulfillment of adult roles tend to increase during adulthood in terms of their average levels.
Beyond providing insights into the general outline of adult personality development, Roberts et al. (2006) found that young adulthood (the period between the ages of 18 and the late 20s) was the most active time in the lifespan for observing average changes, although average differences in personality attributes were observed across the lifespan. Such a result might be surprising in light of the intuition that adolescence is a time of personality change and maturation. However, young adulthood is typically a time in the lifespan that includes a number of life changes in terms of finishing school, starting a career, committing to romantic partnerships, and parenthood (Donnellan, Conger, & Burzette, 2007; Rindfuss, 1991). Finding that young adulthood is an active time for personality development provides circumstantial evidence that adult roles might generate pressures for certain patterns of personality development. Indeed, this is one potential explanation for the maturity principle of personality development.
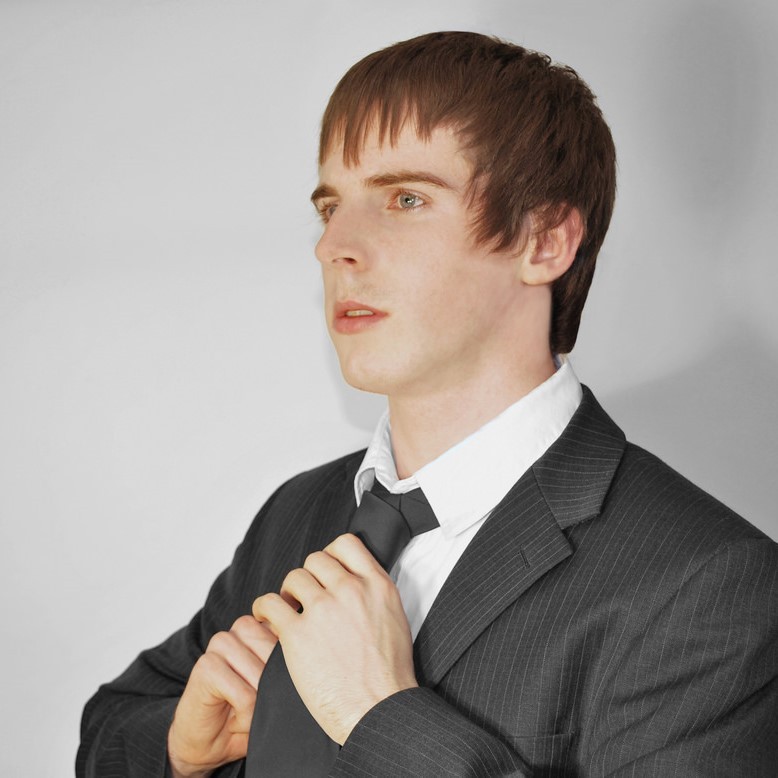
It should be emphasized again that average trends are summaries that do not necessarily apply to all individuals. Some people do not conform to the maturity principle. The possibility of exceptions to general trends is the reason it is necessary to study individual patterns of personality development. The methods for this kind of research are becoming increasingly popular (e.g., Vaidya, Gray, Haig, Mroczek, & Watson, 2008) and existing studies suggest that personality changes differ across people (Roberts & Mroczek, 2008). These new research methods work best when researchers collect more than two waves of longitudinal data covering longer spans of time. This kind of research design is still somewhat uncommon in psychological studies but it will likely characterize the future of research on personality stability.
Differential stability. The evaluation of differential stability requires a longitudinal study. The simplest strategy is to follow a large sample of participants of the same age and measure their personality attributes at two points separated by a meaningful span of time. The researcher then calculates the correlation between scores at the first assessment and scores at the second assessment (a coefficient sometimes called a test-retest correlation or even a stability coefficient). As you know, a correlation coefficient is a numerical summary of the linear association between two variables. Correlations around .1 or –.1 are often called “small” associations, whereas correlations around .50 and –.50 (or larger) are often called “large” associations (Cohen, 1988).
Roberts and DelVecchio (2000) summarized 3,217 test-retest correlations for a wide range of personality attributes reported in 152 longitudinal studies. They used statistical methods to equate the different test-retest correlations to a common interval of about seven years. This allowed them to compare results from studies of differing lengths of time because not all studies followed participants for the same interval of time. Roberts and DelVecchio found that differential stability increased with age. The correlations ranged from about .30 for samples involving young children to about .70 for samples involving older adults. Ferguson (2010) updated and replicated this basic pattern. This pattern of increasing stability with age is called the cumulative continuity principle of personality development (Caspi et al., 2005). This general pattern holds for both women and men and applies to a wide range of different personality attributes ranging from extraversion to openness and curiosity. It is important to emphasize, however, that the observed correlations are never perfect at any age (i.e., the correlations do not reach 1.0). This indicates that personality changes can occur at any time in the lifespan; it just seems that greater inconsistency is observed in childhood and adolescence than in adulthood.
Key Messages So Far
It is useful to summarize the key ideas of this module so far. The starting point was the realization that there are several different ways to define and measure personality stability. Heterotypic stability refers to the consistency of the underlying psychological attribute that may have different behavioral manifestations at different ages. Homotypic stability, on the other hand, refers to the consistency of the same observable manifestations of a personality attribute. This type of stability is commonly studied in the current literature, and absolute and differential stability are a focus on many studies. A consideration of the broad literature on personality stability yields two major conclusions.
- Average levels of personality attributes seem to change in predictable ways across the lifespan in line with maturity principle of personality development. Traits that are correlated with positive outcomes (such as conscientiousness) seem to increase from adolescence to adulthood. This perspective on personality stability is gained from considering absolute stability in the form of average levels of personality attributes at different ages.
- Personality attributes are relatively enduring attributes that become increasingly consistent during adulthood in line with the cumulative continuity principle. This perspective on stability is gained from considering differential stability in the form of test-retest correlations from longitudinal studies.
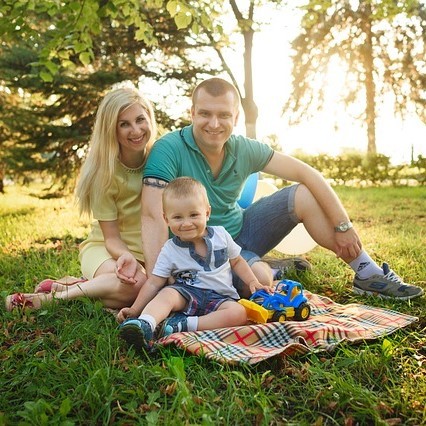
In general, the picture that emerges from the literature is that personality traits are relatively enduring attributes that become more stable from childhood to adulthood. Nonetheless, the stability of personality attributes is not perfect at any period in the lifespan. This is an important conclusion because it challenges two extreme perspectives that have been influential in psychological research. More than 100 years ago, the famous psychologist William James remarked that character (personality) was “set like plaster” for most people by age 30. This perspective implies near perfect stability of personality in adulthood. In contrast, other psychologists have sometimes denied there was any stability to personality at all. Their perspective is that individual thoughts and feelings are simply responses to transitory situational influences that are unlikely to show much consistency across the lifespan. As discussed so far, current research does not support either of these extreme perspectives. Nonetheless, the existence of some degree of stability raises important questions about the exact processes and mechanisms that produce personality stability (and personality change).
The How and Why of Personality Stability and Change: Different Kinds of Interplay Between Individuals and Their Environments
Personality stability is the result of the interplay between the individual and her/his environment. Psychologists use the term person–environment transactions (e.g., Roberts et al., 2008) to capture the mutually transforming interplay between individuals and their contextual circumstances. Several different types of these transactions have been described by psychological researchers. Active person–environment transactions occur when individuals seek out certain kinds of environments and experiences that are consistent with their personality characteristics. Risk-taking individuals may spend their leisure time very differently than more cautious individuals. Some prefer extreme sports whereas others prefer less intense experiences. Reactive person–environment transactions occur when individuals react differently to the same objective situation because of their personalities. A large social gathering represents a psychologically different context to the highly extraverted person compared with the highly introverted person. Evocative person–environment transactions occur whenever individuals draw out or evoke certain kinds of responses from their social environments because of their personality attributes. A warm and secure individual invites different kinds of responses from peers than a cold and aloof individual.
Current researchers make distinctions between the mechanisms likely to produce personality stability and the mechanisms likely to produce changes (Roberts, 2006; Roberts et al., 2008). Brent Roberts coined the helpful acronym ASTMA to aid in remembering many of these mechanisms: Attraction (A), selection (S), manipulation (M), and attrition (A) tend to produce personality stability, whereas transformation (T) explains personality change.
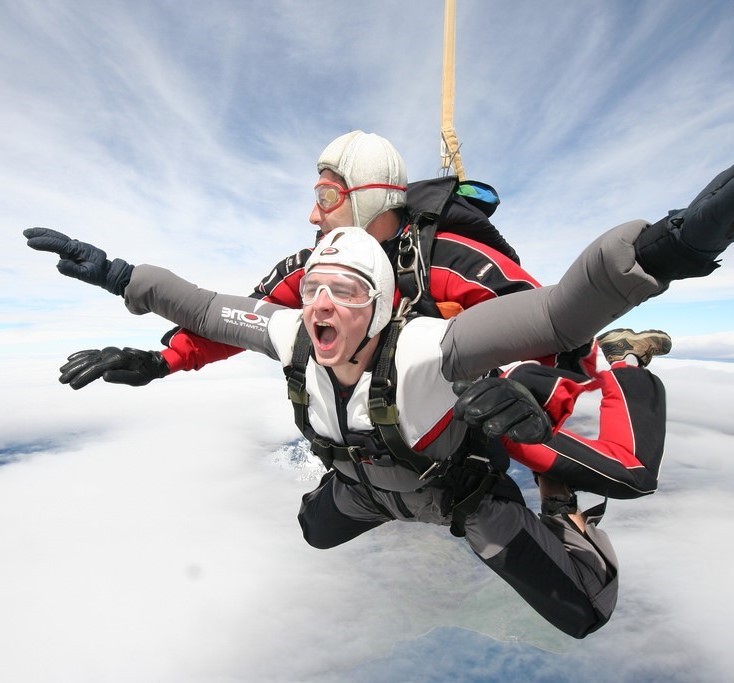
Individuals sometimes select careers, friends, social clubs, and lifestyles because of their personality attributes. This is the active process of attraction—individuals are attracted to environments because of their personality attributes. Situations that match with our personalities seem to feel “right” (e.g., Cesario, Grant, & Higgins, 2004). On the flipside of this process, gatekeepers, such as employers, admissions officers, and even potential relationship partners, often select individuals because of their personalities. Extraverted and outgoing individuals are likely to make better salespeople than quiet individuals who are uncomfortable with social interactions. All in all, certain individuals are “admitted” by gatekeepers into particular kinds of environments because of their personalities. Likewise, individuals with characteristics that are a bad fit with a particular environment may leave such settings or be asked to leave by gatekeepers. A lazy employee will not last long at a demanding job. These examples capture the process of attrition (dropping out). The processes of selection and attrition reflect evocative person–environment transactions. Last, individuals can actively manipulate their environments to match their personalities. An outgoing person will find ways to introduce more social interactions into the workday, whereas a shy individual may shun the proverbial water cooler to avoid having contact with others.
These four processes of attraction, selection, attrition, and manipulation explain how a kind of matching occurs between personality attributes and environmental conditions for many individuals. This positive matching typically produces personality consistency because the “press” of the situation reinforces the attributes of the person. This observation is at the core of the corresponsive principle of personality development (Caspi et al., 2005; Roberts, Caspi, & Moffitt, 2003). Preexisting personality attributes and environmental contexts work in concert to promote personality continuity. The idea is that environments often reinforce those personality attributes that were partially responsible for the initial environmental conditions in the first place. For example, ambitious and confident individuals might be attracted to and selected for more demanding jobs (Roberts et al., 2003). These kinds of jobs often require drive, dedication, and achievement striving thereby accentuating dispositional tendencies toward ambition and confidence.
Additional considerations related to person–environment transactions may help to further explain personality stability. Individuals gain more autonomy to select their own environment as they transition from childhood to adulthood (Scarr & McCartney, 1983). This might help explain why the differential stability of personality attributes increases from adolescence into adulthood. Reactive and evocative person–environment transactions also facilitate personality stability. The overarching idea is that personality attributes shape how individuals respond to situations and shape the kinds of responses individuals elicit from their environments. These responses and reactions can generate self-fulfilling cycles. For example, aggressive individuals seem to interpret ambiguous social cues as threatening (something called a hostile attribution bias or a hostile attribution of intent; see Crick & Dodge, 1996; Orobio de Castro, Veerman, Koops, Bosch, & Monshouwer, 2002). If a stranger runs into you and you spill your hot coffee all over a clean shirt, how do you interpret the situation? Do you believe the other person was being aggressive, or were you just unlucky? A rude, caustic, or violent response might invite a similar response from the individual who ran into you. The basic point is that personality attributes help shape reactions to and responses from the social world, and these processes often (but not always) end up reinforcing dispositional tendencies.
Although a number of mechanisms account for personality continuity by generating a match between the individual’s characteristics and the environment, personality change or transformation is nonetheless possible. Recall that differential stability is not perfect. The simplest mechanism for producing change is a cornerstone of behaviorism: Patterns of behavior that produce positive consequences (pleasure) are repeated, whereas patterns of behavior that produce negative consequences (pain) will diminish (Thorndike, 1933). Social settings may have the power to transform personality if the individual is exposed to different rewards and punishments and the setting places limitations on how a person can reasonably behave (Caspi & Moffitt, 1993). For example, environmental contexts that limit agency and have very clear reward structures such as the military might be particularly powerful contexts for producing lasting personality changes (e.g., Jackson, Thoemmes, Jonkmann, Lüdke, & Trautwein, 2012).
It is also possible that individuals might change their personality attributes by actively striving to change their behaviors and emotional reactions with help from outsiders. This idea lies at the heart of psychotherapy. As it stands, the conditions that produce lasting personality changes are an active area of research. Personality researchers have historically sought to demonstrate the existence of personality stability, and they are now turning their full attention to the conditions that facilitate personality change. There are currently a few examples of interventions that end up producing short-term personality changes (Jackson, Hill, Payne, Roberts, & Stine-Morrow, 2012), and this is an exciting area for future research (Edmonds, Jackson, Fayard, & Roberts, 2008). Insights about personality change are important for creating effective interventions designed to foster positive human development. Finding ways to promote self-control, emotional stability, creativity, and an agreeable disposition would likely lead to improvements for both individuals and society as a whole because these attributes predict a range of consequential life outcomes (Ozer & Benet-Martínez, 2006; Roberts et al., 2007)
Conclusion
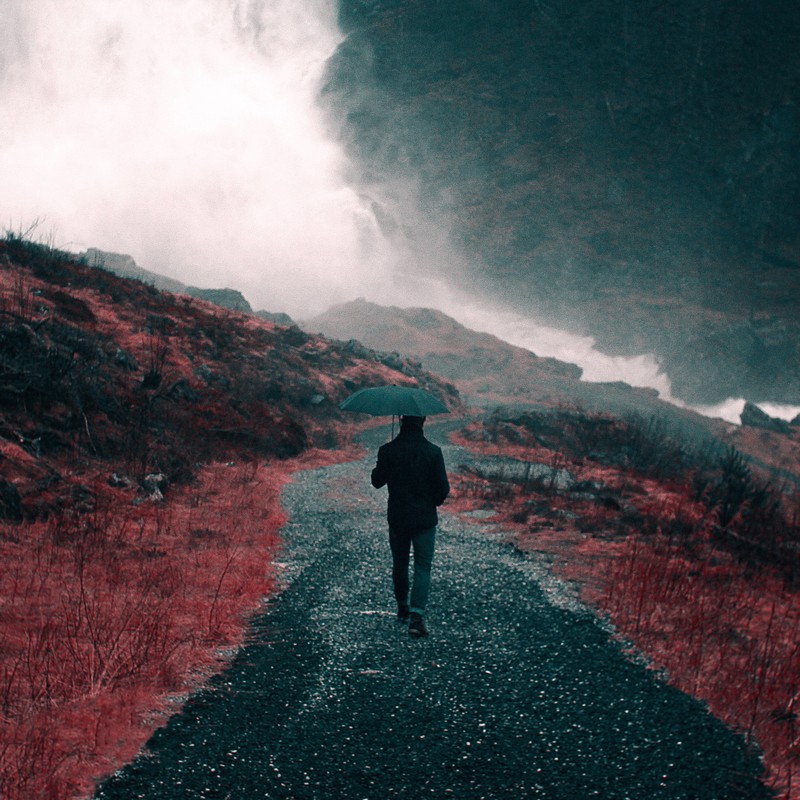
There are multiple ways to evaluate personality stability. The existing evidence suggests that personality attributes are relatively enduring attributes that show predictable average-level changes across the lifespan. Personality stability is produced by a complicated interplay between individuals and their social settings. Many personality attributes are linked to life experiences in a mutually reinforcing cycle: Personality attributes seem to shape environmental contexts, and those contexts often then accentuate and reinforce those very personality attributes. Even so, personality change or transformation is possible because individuals respond to their environments. Individuals may also want to change their personalities. Personality researchers are now beginning to address important questions about the possibility of lasting personality changes through intervention efforts.
[1] Throughout most of this module I will use the term stability to refer to continuity, stability/change, and consistency/inconsistency.
Check Your Knowledge
To help you with your studying, we’ve included some practice questions for this module. These questions do not necessarily address all content in this module. They are intended as practice, and you are responsible for all of the content in this module even if there is no associated practice question. To promote deeper engagement with the material, we encourage you to create some questions of your own for your practice. You can then also return to these self-generated questions later in the course to test yourself.
Vocabulary
- Absolute stability
- Consistency in the level or amount of a personality attribute over time.
- Active person–environment transactions
- The interplay between individuals and their contextual circumstances that occurs whenever individuals play a key role in seeking out, selecting, or otherwise manipulating aspects of their environment.
- Age effects
- Differences in personality between groups of different ages that are related to maturation and development instead of birth cohort differences.
- Attraction
- A connection between personality attributes and aspects of the environment that occurs because individuals with particular traits are drawn to certain environments.
- Attrition
- A connection between personality attributes and aspects of the environment that occurs because individuals with particular traits drop out from certain environments.
- Birth cohort
- Individuals born in a particular year or span of time.
- Cohort effects
- Differences in personality that are related to historical and social factors unique to individuals born in a particular year.
- Corresponsive principle
- The idea that personality traits often become matched with environmental conditions such that an individual’s social context acts to accentuate and reinforce their personality attributes.
- Cross-sectional study/design
- A research design that uses a group of individuals with different ages (and birth cohorts) assessed at a single point in time.
- Cumulative continuity principle
- The generalization that personality attributes show increasing stability with age and experience.
- Differential stability
- Consistency in the rank-ordering of personality across two or more measurement occasions.
- Evocative person–environment transactions
- The interplay between individuals and their contextual circumstances that occurs whenever attributes of the individual draw out particular responses from others in their environment.
- Group level
- A focus on summary statistics that apply to aggregates of individuals when studying personality development. An example is considering whether the average score of a group of 50 year olds is higher than the average score of a group of 21 year olds when considering a trait like conscientiousness.
- Heterotypic stability
- Consistency in the underlying psychological attribute across development regardless of any changes in how the attribute is expressed at different ages.
- Homotypic stability
- Consistency of the exact same thoughts, feelings, and behaviors across development.
- Hostile attribution bias
- The tendency of some individuals to interpret ambiguous social cues and interactions as examples of aggressiveness, disrespect, or antagonism.
- Individual level
- A focus on individual level statistics that reflect whether individuals show stability or change when studying personality development. An example is evaluating how many individuals increased in conscientiousness versus how many decreased in conscientiousness when considering the transition from adolescence to adulthood.
- Longitudinal study/design
- A research design that follows the same group of individuals at multiple time points.
- Manipulation
- A connection between personality attributes and aspects of the environment that occurs whenever individuals with particular traits actively shape their environments.
- Maturity principle
- The generalization that personality attributes associated with the successful fulfillment of adult roles increase with age and experience.
- Person–environment transactions
- The interplay between individuals and their contextual circumstances that ends up shaping both personality and the environment.
- Reactive person–environment transactions
- The interplay between individuals and their contextual circumstances that occurs whenever attributes of the individual shape how a person perceives and responds to their environment.
- Selection
- A connection between personality attributes and aspects of the environment that occurs whenever individuals with particular attributes choose particular kinds of environments.
- Stress reaction
- The tendency to become easily distressed by the normal challenges of life.
- Transformation
- The term for personality changes associated with experience and life events.
References
- Anusic, I., Lucas, R. E., & Donnellan, M. B. (2012). Cross-sectional age differences in personality: Evidence from nationally representative samples from Switzerland and the United States. Journal of Research in Personality, 46, 116–120.
- Caspi, A., & Bem, D. J. (1990). Personality continuity and change across the life course. In L. Pervin (Ed.), Handbook of personality: Theory and research (pp. 549–575). New York, NY: Guilford Press.
- Caspi, A., & Moffitt, T. E. (1993). When do individual differences matter? A paradoxical theory of personality coherence. Psychological Inquiry, 4, 247–271.
- Caspi, A., Bem, D. J., & Elder, G. H., Jr. (1989). Continuities and consequences of interactional styles across the life course. Journal of Personality, 57, 375–406.
- Caspi, A., Roberts, B. W., & Shiner, R. L. (2005). Personality development: Stability and change. Annual Review of Psychology, 56, 453–484.
- Cesario, J., Grant, H., & Higgins, E. T. (2004). Regulatory fit and persuasion: Transfer from “feeling right.” Journal of Personality and Social Psychology, 86, 388–404.
- Cohen, J. (1988). Statistical power analysis for the behavioral sciences (2nd ed.). Hillsdale, NJ: Erlbaum.
- Crick, N. R., & Dodge, K. A. (1996). Social information-processing mechanisms in reactive and proactive aggression. Child Development, 67, 993–1002.
- Donnellan, M. B., Conger, R. D., & Burzette, R. G. (2007). Personality development from late adolescence to young adulthood: Differential stability, normative maturity, and evidence for the maturity-stability hypothesis. Journal of Personality, 75, 237–267.
- Edmonds, G. W., Jackson, J. J., Fayard, J. V., & Roberts, B. W. (2008). Is characters fate, or is there hope to change my personality yet? Social and Personality Compass, 2, 399–413.
- Ferguson, C. J. (2010). A meta-analysis of normal and disordered personality across the lifespan. Journal of Personality and Social Psychology, 98, 659–667.
- Jackson, J. J., Hill, P. L., Payne, B. R., Roberts, B. W., & Stine-Morrow, E. A. L. (2012). Can an old dog learn (and want to experience) new tricks? Cognitive training increases openness to experience in older adults. Psychology and Aging, 27, 286-292.
- Jackson, J. J., Thoemmes, F., Jonkmann, K., Lüdtke, O., & Trautwein, U. (2012). Military training and personality trait development: Does the military make the man, or does the man make the military? Psychological Science, 23, 270–277.
- John, O. P., Naumann, L. P., & Soto, C. J. (2008). Paradigm shift to the integrative Big Five trait taxonomy: History, measurement, and conceptual issues. In O. P. John, R. W. Robins, and L. A. Pervin (Eds.), Handbook of personality: Theory and Research (3rd ed., pp. 114–158). New York, NY: Guilford Press.
- Kotov, R., Gamez, W., Schmidt, F., & Watson, D. (2010). Linking “big” personality traits to anxiety, depressive, and substance use disorders: A meta-analysis. Psychological Bulletin 136, 768–821.
- Lucas, R. E., & Donnellan, M. B. (2011). Personality development across the lifespan: Longitudinal analyses with a national sample from Germany. Journal of Personality and Social Psychology, 101, 847–861.
- Lucas, R. E., & Donnellan, M. B. (2009). Age differences in personality: Evidence from a nationally representative sample of Australians. Developmental Psychology, 45, 1353–1363.
- McCrae, R. R., & Costa, P. T., Jr. (2003). Personality in adulthood: A five-factor theory perspective (2nd ed.). New York, NY: Guilford Press.
- Miller, J. D., & Lynam, D. (2001). Structural models of personality and their relation to antisocial behavior: A meta-analytic review. Criminology, 39, 765–798.
- Mroczek, D. K., & Spiro, A., III (2007). Personality change influences mortality in older men. Psychological Science, 18, 371–376.
- Orobio de Castro, B., Veerman, J. W., Koops, W., Bosch, J. D., & Monshouwer, H. J. (2002). Hostile attribution of intent and aggressive behavior: A meta-analysis. Child Development, 73, 916–934.
- Ozer, D. J., & Benet-Martínez, V. (2006). Personality and the prediction of consequential outcomes. Annual Review of Psychology, 57, 401–421.
- Rindfuss, R. R. (1991). The young adult years: Diversity, structural change, and fertility. Demography, 28, 493–512.
- Roberts, B. W. (2006). Personality development and organizational behavior. Research in Organizational Behavior, 27, 1–40.
- Roberts, B. W., & DelVecchio, W. F. (2000). The rank-order consistency of personality traits from childhood to old age: A quantitative review of longitudinal studies. Psychological Bulletin, 126, 3–25.
- Roberts, B. W., & Mroczek, D. (2008). Personality trait change in adulthood. Current Directions in Psychological Science, 17, 31–35.
- Roberts, B. W., Caspi, A., & Moffitt, T. (2003). Work experiences and personality development in young adulthood. Journal of Personality and Social Psychology, 84, 582–593.
- Roberts, B. W., Kuncel, N., Shiner, R. N., Caspi, A., & Goldberg, L. (2007). The power of personality: The comparative validity of personality traits, socioeconomic status, and cognitive ability for predicting important life outcomes. Perspectives in Psychological Science, 2, 313–345.
- Roberts, B. W., Kuncel, N., Shiner, R. N., Caspi, A., & Goldberg, L. (2007). The power of personality: The comparative validity of personality traits, socioeconomic status, and cognitive ability for predicting important life outcomes. Perspectives in Psychological Science, 2, 313–345.
- Roberts, B. W., Walton, K., & Viechtbauer, W. (2006). Patterns of mean-level change in personality traits across the life course: A meta-analysis of longitudinal studies. Psychological Bulletin, 132, 1–25.
- Roberts, B. W., Wood, D., & Caspi, A. (2008). Personality Development. In O. P. John, R. W. Robins, & L. A. Pervin (Eds.), Handbook of personality: theory and research (3rd ed., pp. 375–398). New York, NY: Guilford Press.
- Scarr, S., & McCartney, K. (1983). How people make their own environments: A theory of genotype → environment effects. Child Development, 54, 424–435.
- Soto, C. J., John, O. P., Gosling, S. D., & Potter, J. (2011). Age differences in personality traits from 10 to 65: Big Five domains and facets in a large cross-sectional sample. Journal of Personality and Social Psychology, 100, 330–348.
- Specht, J., Egloff, B., & Schmukle, S. C. (2011). Stability and change of personality across the life course: The impact of age and major life events on mean-level and rank-order stability of the Big Five. Journal of Personality and Social Psychology, 101, 862–882.
- Srivastava, S., John, O. P., Gosling, S. D., & Potter, J. (2003). Development of personality in early and middle adulthood: Set like plaster or persistent change? Journal of Personality and Social Psychology, 84, 1041–1053.
- Terracciano, A., McCrae, R. R., Brant, L. J., & Costa, P. T., Jr. (2005). Hierarchical linear modeling analyses of the NEO-PI-R scales in the Baltimore Longitudinal Study of Aging. Psychology and Aging, 20, 493–506.
- Thorndike, E. L. (1933). A proof of the law of effect. Science, 77, 173–175.
- Vaidya, J. G., Gray, E. K., Haig, J. R., Mroczek, D. K., & Watson (2008). Differential stability and individual growth trajectories of Big Five and affective traits during young adulthood. Journal of Personality, 76, 267–304.
- Wortman, J., Lucas, R. E., & Donnellan, M. B. (in press). Stability and change in the Big Five personality domains: Evidence from a longitudinal study of Australians. Psychology and Aging.
How to cite this Chapter using APA Style:
Donnellan, M. B. (2019). Personality stability and change. Adapted for use by Queen's University. Original chapter in R. Biswas-Diener & E. Diener (Eds), Noba textbook series: Psychology. Champaign, IL: DEF publishers. Retrieved from http://noba.to/sjvtxbwd
Copyright and Acknowledgment:
This material is licensed under the Creative Commons Attribution-NonCommercial-ShareAlike 4.0 International License. To view a copy of this license, visit: http://creativecommons.org/licenses/by-nc-sa/4.0/deed.en_US.
This material is attributed to the Diener Education Fund (copyright © 2018) and can be accessed via this link: http://noba.to/sjvtxbwd.
Additional information about the Diener Education Fund (DEF) can be accessed here.
Original chapter by Ian Weaver adapted by Queen's University Psychology Department
This Open Access chapter was originally written for the NOBA project. Information on the NOBA project can be found below.
Early life experiences exert a profound and long-lasting influence on physical and mental health throughout life. The efforts to identify the primary causes of this have significantly benefited from studies of the epigenome—a dynamic layer of information associated with DNA that differs between individuals and can be altered through various experiences and environments. The epigenome has been heralded as a key “missing piece” of the etiological puzzle for understanding how development of psychological disorders may be influenced by the surrounding environment, in concordance with the genome. Understanding the mechanisms involved in the initiation, maintenance, and heritability of epigenetic states is thus an important aspect of research in current biology, particularly in the study of learning and memory, emotion, and social behavior in humans. Moreover, epigenetics in psychology provides a framework for understanding how the expression of genes is influenced by experiences and the environment to produce individual differences in behavior, cognition, personality, and mental health. In this module, we survey recent developments revealing epigenetic aspects of mental health and review some of the challenges of epigenetic approaches in psychology to help explain how nurture shapes nature.
Learning Objectives
- Explain what the term epigenetics means and the molecular machinery involved.
- Name and discuss important neural and developmental pathways that are regulated by epigenetic factors, and provide examples of epigenetic effects on personality traits and cognitive behavior.
- Understand how misregulation of epigenetic mechanisms can lead to disease states, and be able to discuss examples.
- Recognize how epigenetic machinery can be targets for therapeutic agents, and discuss examples.
Introduction
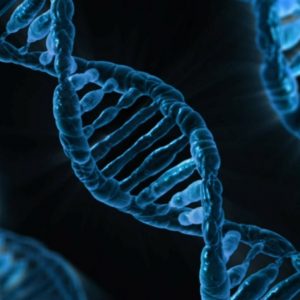
Early childhood is not only a period of physical growth; it is also a time of mental development related to changes in the anatomy, physiology, and chemistry of the nervous system that influence mental health throughout life. Cognitive abilities associated with learning and memory, reasoning, problem solving, and developing relationships continue to emerge during childhood. Brain development is more rapid during this critical or sensitive period than at any other, with more than 700 neural connections created each second. Herein, complex gene–environment interactions (or genotype–environment interactions, G×E) serve to increase the number of possible contacts between neurons, as they hone their adult synaptic properties and excitability. Many weak connections form to different neuronal targets; subsequently, they undergo remodeling in which most connections vanish and a few stable connections remain. These structural changes (or plasticity) may be crucial for the development of mature neural networks that support emotional, cognitive, and social behavior. The generation of different morphology, physiology, and behavioral outcomes from a single genome in response to changes in the environment forms the basis for “phenotypic plasticity,” which is fundamental to the way organisms cope with environmental variation, navigate the present world, and solve future problems.
The challenge for psychology has been to integrate findings from genetics and environmental (social, biological, chemical) factors, including the quality of infant–mother attachments, into the study of personality and our understanding of the emergence of mental illness. These studies have demonstrated that common DNA sequence variation and rare mutations account for only a small fraction (1%–2%) of the total risk for inheritance of personality traits and mental disorders (Dick, Riley, & Kendler, 2010; Gershon, Alliey-Rodriguez, & Liu, 2011). Additionally, studies that have attempted to examine the mechanisms and conditions under which DNA sequence variation influences brain development and function have been confounded by complex cause-and-effect relationships (Petronis, 2010). The large unaccounted heritability of personality traits and mental health suggests that additional molecular and cellular mechanisms are involved.
Epigenetics has the potential to provide answers to these important questions and refers to the transmission of phenotype in terms of gene expression in the absence of changes in DNA sequence—hence the name epi- (Greek: επί- over, above) genetics (Waddington, 1942; Wolffe & Matzke, 1999). The advent of high-throughput techniques such as sequencing-based approaches to study the distributions of regulators of gene expression throughout the genome led to the collective description of the “epigenome.” In contrast to the genome sequence, which is static and the same in almost all cells, the epigenome is highly dynamic, differing among cell types, tissues, and brain regions (Gregg et al., 2010). Recent studies have provided insights into epigenetic regulation of developmental pathways in response to a range of external environmental factors (Dolinoy, Weidman, & Jirtle, 2007). These environmental factors during early childhood and adolescence can cause changes in expression of genes conferring risk of mental health and chronic physical conditions. Thus, the examination of genetic–epigenetic–environment interactions from a developmental perspective may determine the nature of gene misregulation in psychological disorders.
This module will provide an overview of the main components of the epigenome and review themes in recent epigenetic research that have relevance for psychology, to form the biological basis for the interplay between environmental signals and the genome in the regulation of individual differences in physiology, emotion, cognition, and behavior.
Molecular control of gene expression: the dynamic epigenome
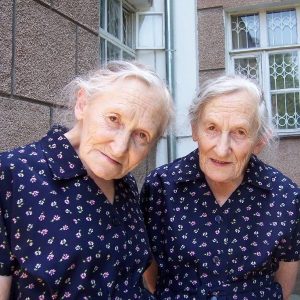
Almost all the cells in our body are genetically identical, yet our body generates many different cell types, organized into different tissues and organs, and expresses different proteins. Within each type of mammalian cell, about 2 meters of genomic DNA is divided into nuclear chromosomes. Yet the nucleus of a human cell, which contains the chromosomes, is only about 2 μm in diameter. To achieve this 1,000,000-fold compaction, DNA is wrapped around a group of 8 proteins called histones. This combination of DNA and histone proteins forms a special structure called a “nucleosome,” the basic unit of chromatin, which represents a structural solution for maintaining and accessing the tightly compacted genome. These factors alter the likelihood that a gene will be expressed or silenced. Cellular functions such as gene expression, DNA replication, and the generation of specific cell types are therefore influenced by distinct patterns of chromatin structure, involving covalent modification of both histones (Kadonaga, 1998) and DNA (Razin, 1998).
Importantly, epigenetic variation also emerges across the lifespan. For example, although identical twins share a common genotype and are genetically identical and epigenetically similar when they are young, as they age they become more dissimilar in their epigenetic patterns and often display behavioral, personality, or even physical differences, and have different risk levels for serious illness. Thus, understanding the structure of the nucleosome is key to understanding the precise and stable control of gene expression and regulation, providing a molecular interface between genes and environmentally induced changes in cellular activity.
The primary epigenetic mark: DNA modification
DNA methylation is the best-understood epigenetic modification influencing gene expression. DNA is composed of four types of naturally occurring nitrogenous bases: adenine (A), thymine (T), guanine (G), and cytosine (C). In mammalian genomes, DNA methylation occurs primarily at cytosine residues in the context of cytosines that are followed by guanines (CpG dinucleotides), to form 5-methylcytosine in a cell-specific pattern (Goll & Bestor, 2005; Law & Jacobsen, 2010; Suzuki & Bird, 2008). The enzymes that perform DNA methylation are called DNA methyltransferases (DNMTs), which catalyze the transfer of a methyl group to the cytosine (Adams, McKay, Craig, & Burdon, 1979). These enzymes are all expressed in the central nervous system and are dynamically regulated during development (Feng, Chang, Li, & Fan, 2005; Goto et al., 1994). The effect of DNA methylation on gene function varies depending on the period of development during which the methylation occurs and location of the methylated cytosine. Methylation of DNA in gene regulatory regions (promoter and enhancer regions) usually results in gene silencing and reduced gene expression (Ooi, O’Donnell, & Bestor, 2009; Suzuki & Bird, 2008; Sutter and Doerfler, 1980; Vardimon et al., 1982). This is a powerful regulatory mechanism that ensures that genes are expressed only when needed. Thus DNA methylation may broadly impact human brain development, and age-related misregulation of DNA methylation is associated with the molecular pathogenesis of neurodevelopmental disorders.
Histone modification and the histone code
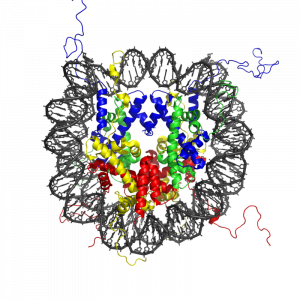
The modification of histone proteins comprises an important epigenetic mark related to gene expression. One of the most thoroughly studied modifications is histone acetylation, which is associated with gene activation and increased gene expression (Wade, Pruss, & Wolffe, 1997). Acetylation on histone tails is mediated by the opposing enzymatic activities of histone acetyltransferases (HATs) and histone deacetylases (HDACs) (Kuo & Allis, 1998). For example, acetylation of histone in gene regulatory regions by HAT enzymes is generally associated with DNA demethylation, gene activation, and increased gene expression (Hong, Schroth, Matthews, Yau, & Bradbury, 1993; Sealy & Chalkley, 1978). On the other hand, removal of the acetyl group (deacetylation) by HDAC enzymes is generally associated with DNA methylation, gene silencing, and decreased gene expression (Davie & Chadee, 1998). The relationship between patterns of histone modifications and gene activity provides evidence for the existence of a “histone code” for determining cell-specific gene expression programs (Jenuwein & Allis, 2001). Interestingly, recent research using animal models has demonstrated that histone modifications and DNA methylation of certain genes mediates the long-term behavioral effects of the level of care experienced during infancy.
Early childhood experience
The development of an individual is an active process of adaptation that occurs within a social and economic context. For example, the closeness or degree of positive attachment of the parent (typically mother)–infant bond and parental investment (including nutrient supply provided by the parent) that define early childhood experience also program the development of individual differences in stress responses in the brain, which then affect memory, attention, and emotion. In terms of evolution, this process provides the offspring with the ability to physiologically adjust gene expression profiles contributing to the organization and function of neural circuits and molecular pathways that support (1) biological defensive systems for survival (e.g., stress resilience), (2) reproductive success to promote establishment and persistence in the present environment, and (3) adequate parenting in the next generation (Bradshaw, 1965).
Parental investment and programming of stress responses in the offspring
The most comprehensive study to date of variations in parental investment and epigenetic inheritance in mammals is that of the maternally transmitted responses to stress in rats. In rat pups, maternal nurturing (licking and grooming) during the first week of life is associated with long-term programming of individual differences in stress responsiveness, emotionality, cognitive performance, and reproductive behavior (Caldji et al., 1998; Francis, Diorio, Liu, & Meaney, 1999; Liu et al., 1997; Myers, Brunelli, Shair, Squire, & Hofer, 1989; Stern, 1997). In adulthood, the offspring of mothers that exhibit increased levels of pup licking and grooming over the first week of life show increased expression of the glucocorticoid receptor in the hippocampus (a brain structure associated with stress responsivity as well as learning and memory) and a lower hormonal response to stress compared with adult animals reared by low licking and grooming mothers (Francis et al., 1999; Liu et al., 1997). Moreover, rat pups that received low levels of maternal licking and grooming during the first week of life showed decreased histone acetylation and increased DNA methylation of a neuron-specific promoter of the glucocorticoid receptor gene (Weaver et al., 2004). The expression of this gene is then reduced, the number of glucocorticoid receptors in the brain is decreased, and the animals show a higher hormonal response to stress throughout their life. The effects of maternal care on stress hormone responses and behaviour in the offspring can be eliminated in adulthood by pharmacological treatment (HDAC inhibitor trichostatin A, TSA) or dietary amino acid supplementation (methyl donor L-methionine), treatments that influence histone acetylation, DNA methylation, and expression of the glucocorticoid receptor gene (Weaver et al., 2004; Weaver et al., 2005). This series of experiments shows that histone acetylation and DNA methylation of the glucocorticoid receptor gene promoter is a necessary link in the process leading to the long-term physiological and behavioral sequelae of poor maternal care. This points to a possible molecular target for treatments that may reverse or ameliorate the traces of childhood maltreatment.
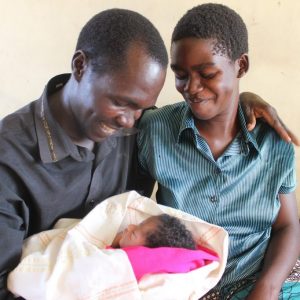
Several studies have attempted to determine to what extent the findings from model animals are transferable to humans. Examination of post-mortem brain tissue from healthy human subjects found that the human equivalent of the glucocorticoid receptor gene promoter (NR3C1 exon 1F promoter) is also unique to the individual (Turner, Pelascini, Macedo, & Muller, 2008). A similar study examining newborns showed that methylation of the glucocorticoid receptor gene promoter maybe an early epigenetic marker of maternal mood and risk of increased hormonal responses to stress in infants 3 months of age (Oberlander et al., 2008). Although further studies are required to examine the functional consequence of this DNA methylation, these findings are consistent with our studies in the neonate and adult offspring of low licking and grooming mothers that show increased DNA methylation of the promoter of the glucocorticoid receptor gene, decreased glucocorticoid receptor gene expression, and increased hormonal responses to stress (Weaver et al., 2004). Examination of brain tissue from suicide victims found that the human glucocorticoid receptor gene promoter is also more methylated in the brains of individuals who had experienced maltreatment during childhood (McGowan et al., 2009). These finding suggests that DNA methylation mediates the effects of early environment in both rodents and humans and points to the possibility of new therapeutic approaches stemming from translational epigenetic research. Indeed, similar processes at comparable epigenetic labile regions could explain why the adult offspring of high and low licking/grooming mothers exhibit widespread differences in hippocampal gene expression and cognitive function (Weaver, Meaney, & Szyf, 2006).
However, this type of research is limited by the inaccessibility of human brain samples. The translational potential of this finding would be greatly enhanced if the relevant epigenetic modification can be measured in an accessible tissue. Examination of blood samples from adult patients with bipolar disorder, who also retrospectively reported on their experiences of childhood abuse and neglect, found that the degree of DNA methylation of the human glucocorticoid receptor gene promoter was strongly positively related to the reported experience of childhood maltreatment decades earlier. For a relationship between a molecular measure and reported historical exposure, the effects size is extraordinarily large. This opens a range of new possibilities: given the large effect size and consistency of this association, measurement of the GR promoter methylation may effectively become a blood test measuring the physiological traces left on the genome by early experiences. Although this blood test cannot replace current methods of diagnosis, this unique and addition information adds to our knowledge of how disease may arise and be manifested throughout life. Near-future research will examine whether this measure adds value over and above simple reporting of early adversities when it comes to predicting important outcomes, such as response to treatment or suicide.
Child nutrition and the epigenome
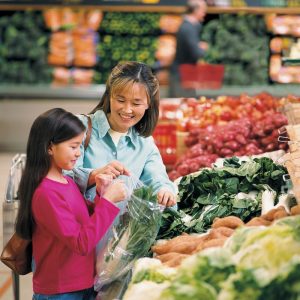
The old adage “you are what you eat” might be true on more than just a physical level: The food you choose (and even what your parents and grandparents chose) is reflected in your own personal development and risk for disease in adult life (Wells, 2003). Nutrients can reverse or change DNA methylation and histone modifications, thereby modifying the expression of critical genes associated with physiologic and pathologic processes, including embryonic development, aging, and carcinogenesis. It appears that nutrients can influence the epigenome either by directly inhibiting enzymes that catalyze DNA methylation or histone modifications, or by altering the availability of substrates necessary for those enzymatic reactions. For example, rat mothers fed a diet low in methyl group donors during pregnancy produce offspring with reduced DNMT-1 expression, decreased DNA methylation, and increased histone acetylation at promoter regions of specific genes, including the glucocorticoid receptor, and increased gene expression in the liver of juvenile offspring (Lillycrop, Phillips, Jackson, Hanson, & Burdge, 2005) and adult offspring (Lillycrop et al., 2007). These data suggest that early life nutrition has the potential to influence epigenetic programming in the brain not only during early development but also in adult life, thereby modulating health throughout life. In this regard, nutritional epigenetics has been viewed as an attractive tool to prevent pediatric developmental diseases and cancer, as well as to delay aging-associated processes.
The best evidence relating to the impact of adverse environmental conditions development and health comes from studies of the children of women who were pregnant during two civilian famines of World War II: the Siege of Leningrad (1941–44) (Bateson, 2001) and the Dutch Hunger Winter (1944–1945) (Stanner et al., 1997). In the Netherlands famine, women who were previously well nourished were subjected to low caloric intake and associated environmental stressors. Women who endured the famine in the late stages of pregnancy gave birth to smaller babies (Lumey & Stein, 1997) and these children had an increased risk of insulin resistance later in life (Painter, Roseboom, & Bleker, 2005). In addition, offspring who were starved prenatally later experienced impaired glucose tolerance in adulthood, even when food was more abundant (Stanner et al., 1997). Famine exposure at various stages of gestation was associated with a wide range of risks such as increased obesity, higher rates of coronary heart disease, and lower birth weight (Lumey & Stein, 1997). Interestingly, when examined 60 years later, people exposed to famine prenatally showed reduced DNA methylation compared with their unexposed same-sex siblings (Heijmans et al., 2008).
Epigenetic regulation of learning and memory
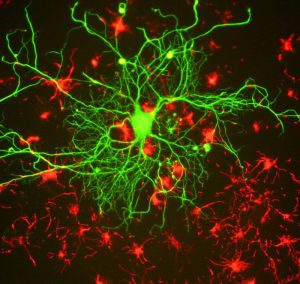
Memories are recollections of actual events stored within our brains. But how is our brain able to form and store these memories? Epigenetic mechanisms influence genomic activities in the brain to produce long-term changes in synaptic signaling, organization, and morphology, which in turn support learning and memory (Day & Sweatt, 2011).
Neuronal activity in the hippocampus of mice is associated with changes in DNA methylation (Guo et al., 2011), and disruption to genes encoding the DNA methylation machinery cause learning and memory impairments (Feng et al., 2010). DNA methylation has also been implicated in the maintenance of long-term memories, as pharmacological inhibition of DNA methylation and impaired memory (Day & Sweatt, 2011; Miller et al., 2010). These findings indicate the importance of DNA methylation in mediating synaptic plasticity and cognitive functions, both of which are disturbed in psychological illness.
Changes in histone modifications can also influence long-term memory formation by altering chromatin accessibility and the expression of genes relevant to learning and memory. Memory formation and the associated enhancements in synaptic transmission are accompanied by increases in histone acetylation (Guan et al., 2002) and alterations in histone methylation (Schaefer et al., 2009), which promote gene expression. Conversely, a neuronal increase in histone deacetylase activity, which promotes gene silencing, results in reduced synaptic plasticity and impairs memory (Guan et al., 2009). Pharmacological inhibition of histone deacetylases augments memory formation (Guan et al., 2009; Levenson et al., 2004), further suggesting that histone (de)acetylation regulates this process.
In humans genetic defects in genes encoding the DNA methylation and chromatin machinery exhibit profound effects on cognitive function and mental health (Jiang, Bressler, & Beaudet, 2004). The two best-characterized examples are Rett syndrome (Amir et al., 1999) and Rubinstein-Taybi syndrome (RTS) (Alarcon et al., 2004), which are profound intellectual disability disorders. Both MECP2 and CBP are highly expressed in neurons and are involved in regulating neural gene expression (Chen et al., 2003; Martinowich et al., 2003).
Rett syndrome patients have a mutation in their DNA sequence in a gene called MECP2. MECP2 plays many important roles within the cell: One of these roles is to read the DNA sequence, checking for DNA methylation, and to bind to areas that contain methylation, thereby preventing the wrong proteins from being present. Other roles for MECP2 include promoting the presence of particular, necessary, proteins, ensuring that DNA is packaged properly within the cell and assisting with the production of proteins. MECP2 function also influences gene expression that supports dendritic and synaptic development and hippocampus-dependent memory (Li, Zhong, Chau, Williams, & Chang, 2011; Skene et al., 2010). Mice with altered MECP2 expression exhibit genome-wide increases in histone acetylation, neuron cell death, increased anxiety, cognitive deficits, and social withdrawal (Shahbazian et al., 2002). These findings support a model in which DNA methylation and MECP2 constitute a cell-specific epigenetic mechanism for regulation of histone modification and gene expression, which may be disrupted in Rett syndrome.
RTS patients have a mutation in their DNA sequence in a gene called CBP. One of these roles of CBP is to bind to specific histones and promote histone acetylation, thereby promoting gene expression. Consistent with this function, RTS patients exhibit a genome-wide decrease in histone acetylation and cognitive dysfunction in adulthood (Kalkhoven et al., 2003). The learning and memory deficits are attributed to disrupted neural plasticity (Korzus, Rosenfeld, & Mayford, 2004). Similar to RTS in humans, mice with a mutation of CBP perform poorly in cognitive tasks and show decreased genome-wide histone acetylation (for review, see Josselyn, 2005). In the mouse brain CBP was found to act as an epigenetic switch to promote the birth of new neurons in the brain. Interestingly, this epigenetic mechanism is disrupted in the fetal brains of mice with a mutation of CBP, which, as pups, exhibit early behavioral deficits following removal and separation from their mother (Wang et al., 2010). These findings provide a novel mechanism whereby environmental cues, acting through histone modifying enzymes, can regulate epigenetic status and thereby directly promote neurogenesis, which regulates neurobehavioral development.
Together, these studies demonstrate that misregulation of epigenetic modifications and their regulatory enzymes is capable of orchestrating prominent deficits in neuronal plasticity and cognitive function. Knowledge from these studies may provide greater insight into other mental disorders such as depression and suicidal behaviors.
Epigenetic mechanisms in psychological disorders
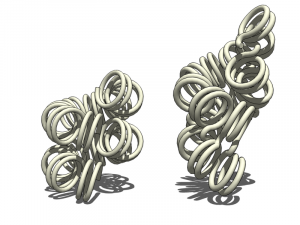
Epigenome-wide studies have identified several dozen sites with DNA methylation alterations in genes involved in brain development and neurotransmitter pathways, which had previously been associated with mental illness (Mill et al., 2008). These disorders are complex and typically start at a young age and cause lifelong disability. Often, limited benefits from treatment make these diseases some of the most burdensome disorders for individuals, families, and society. It has become evident that the efforts to identify the primary causes of complex psychiatric disorders may significantly benefit from studies linking environmental effects with changes observed within the individual cells.
Epigenetic events that alter chromatin structure to regulate programs of gene expression have been associated with depression-related behavior and action of antidepressant medications, with increasing evidence for similar mechanisms occurring in post-mortem brains of depressed individuals. In mice, social avoidance resulted in decreased expression of hippocampal genes important in mediating depressive responses (Tsankova et al., 2006). Similarly, chronic social defeat stress was found to decrease expression of genes implicated in normal emotion processing (Lutter et al., 2008). Consistent with these findings, levels of histone markers of increased gene expression were down regulated in human post-mortem brain samples from individuals with a history of clinical depression (Covington et al., 2009).
Administration of antidepressants increased histone markers of increased gene expression and reversed the gene repression induced by defeat stress (Lee, Wynder, Schmidt, McCafferty, & Shiekhattar, 2006; Tsankova et al., 2006; Wilkinson et al., 2009). These results provide support for the use of HDAC inhibitors against depression. Accordingly, several HDAC inhibitors have been found to exert antidepressant effects by each modifying distinct cellular targets (Cassel et al., 2006; Schroeder, Lin, Crusio, & Akbarian, 2007).
There is also increasing evidence that aberrant gene expression resulting from altered epigenetic regulation is associated with the pathophysiology of suicide (McGowan et al., 2008; Poulter et al., 2008). Thus, it is tempting to speculate that there is an epigenetically determined reduced capacity for gene expression, which is required for learning and memory, in the brains of suicide victims.
Epigenetic strategy to understanding gene-environment interactions
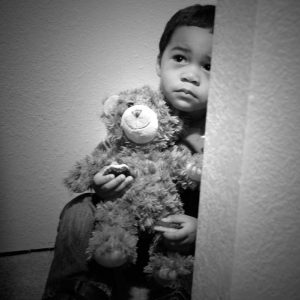
While the cellular and molecular mechanisms that influence on physical and mental health have long been a central focus of neuroscience, only in recent years has attention turned to the epigenetic mechanisms behind the dynamic changes in gene expression responsible for normal cognitive function and increased risk for mental illness. The links between early environment and epigenetic modifications suggest a mechanism underlying gene-environment interactions. Early environmental adversity alone is not a sufficient cause of mental illness, because many individuals with a history of severe childhood maltreatment or trauma remain healthy. It is increasingly becoming evident that inherited differences in the segments of specific genes may moderate the effects of adversity and determine who is sensitive and who is resilient through a gene-environment interplay. Genes such as the glucocorticoid receptor appear to moderate the effects of childhood adversity on mental illness. Remarkably, epigenetic DNA modifications have been identified that may underlie the long-lasting effects of environment on biological functions. This new epigenetic research is pointing to a new strategy to understanding gene-environment interactions.
The next decade of research will show if this potential can be exploited in the development of new therapeutic options that may alter the traces that early environment leaves on the genome. However, as discussed in this module, the epigenome is not static and can be molded by developmental signals, environmental perturbations, and disease states, which present an experimental challenge in the search for epigenetic risk factors in psychological disorders (Rakyan, Down, Balding, & Beck, 2011). The sample size and epigenomic assay required is dependent on the number of tissues affected, as well as the type and distribution of epigenetic modifications. The combination of genetic association maps studies with epigenome-wide developmental studies may help identify novel molecular mechanisms to explain features of inheritance of personality traits and transform our understanding of the biological basis of psychology. Importantly, these epigenetic studies may lead to identification of novel therapeutic targets and enable the development of improved strategies for early diagnosis, prevention, and better treatment of psychological and behavioral disorders.
A "Big Picture" Insight into Epi-Genetics
Epigentics is a content area where students sometimes feel apprehensive, especially if they do not have a background in biology. This course will teach you all required biology content expected for this class. To help get you excited about the importance of this research field, please watch the following video. It provides insights into why it is so important for behavioural scientists to understand, at least on a surface level, about the field of epigenetics. After you watch it, please be sure to go through this module again. This video is not intended as a replacement for the content in this module, but rather is intended to serve as a big-picture framework for you to better understand the content in this module.
Check Your Knowledge
To help you with your studying, we’ve included some practice questions for this module. These questions do not necessarily address all content in this module. They are intended as practice, and you are responsible for all of the content in this module even if there is no associated practice question. To promote deeper engagement with the material, we encourage you to create some questions of your own for your practice. You can then also return to these self-generated questions later in the course to test yourself.
Vocabulary
- DNA methylation
- Covalent modifications of mammalian DNA occurring via the methylation of cytosine, typically in the context of the CpG dinucleotide.
- DNA methyltransferases (DNMTs)
- Enzymes that establish and maintain DNA methylation using methyl-group donor compounds or cofactors. The main mammalian DNMTs are DNMT1, which maintains methylation state across DNA replication, and DNMT3a and DNMT3b, which perform de novo methylation.
- Epigenetics
- The study of heritable changes in gene expression or cellular phenotype caused by mechanisms other than changes in the underlying DNA sequence. Epigenetic marks include covalent DNA modifications and posttranslational histone modifications.
- Epigenome
- The genome-wide distribution of epigenetic marks.
- Gene
- A specific deoxyribonucleic acid (DNA) sequence that codes for a specific polypeptide or protein or an observable inherited trait.
- Genome-wide association study (GWAS)
- A study that maps DNA polymorphisms in affected individuals and controls matched for age, sex, and ethnic background with the aim of identifying causal genetic variants.
- Genotype
- The DNA content of a cell’s nucleus, whether a trait is externally observable or not.
- Histone acetyltransferases (HATs) and histone deacetylases (HDACs)
- HATs are enzymes that transfer acetyl groups to specific positions on histone tails, promoting an “open” chromatin state and transcriptional activation. HDACs remove these acetyl groups, resulting in a “closed” chromatin state and transcriptional repression.
- Histone modifications
- Posttranslational modifications of the N-terminal “tails” of histone proteins that serve as a major mode of epigenetic regulation. These modifications include acetylation, phosphorylation, methylation, sumoylation, ubiquitination, and ADP-ribosylation.
- Identical twins
- Two individual organisms that originated from the same zygote and therefore are genetically identical or very similar. The epigenetic profiling of identical twins discordant for disease is a unique experimental design as it eliminates the DNA sequence-, age-, and sex-differences from consideration.
- Phenotype
- The pattern of expression of the genotype or the magnitude or extent to which it is observably expressed—an observable characteristic or trait of an organism, such as its morphology, development, biochemical or physiological properties, or behavior.
References
- Adams, R. L., McKay, E. L., Craig, L. M., & Burdon, R. H. (1979). Mouse DNA methylase: methylation of native DNA. Biochimica et Biophysica Acta, 561(2), 345–357.
- Alarcon, J. M., Malleret, G., Touzani, K., Vronskaya, S., Ishii, S., Kandel, E. R., & Barco, A. (2004). Chromatin acetylation, memory, and LTP are impaired in CBP+/- mice: a model for the cognitive deficit in Rubinstein-Taybi syndrome and its amelioration. Neuron, 42(6), 947–959. doi: 10.1016/j.neuron.2004.05.021, S0896627304003022 [pii]
- Amir, R. E., Van den Veyver, I. B., Wan, M., Tran, C. Q., Francke, U., & Zoghbi, H. Y. (1999). Rett syndrome is caused by mutations in X-linked MECP2, encoding methyl-CpG-binding protein 2. Nature Genetics, 23(2), 185–188.
- Bateson, P. (2001). Fetal experience and good adult design. International Journal of Epidemiology, 30(5), 928–934.
- Bradshaw, A.D. (1965). Evolutionary significance of phenotypic plasticity in plants. Advances in Genetics, 13, 115–155.
- Caldji, C., Tannenbaum, B., Sharma, S., Francis, D., Plotsky, P. M., & Meaney, M. J. (1998). Maternal care during infancy regulates the development of neural systems mediating the expression of fearfulness in the rat. Proceedings of the National Academy of Sciences U S A, 95(9), 5335–5340.
- Cassel, S., Carouge, D., Gensburger, C., Anglard, P., Burgun, C., Dietrich, J. B., . . . Zwiller, J. (2006). Fluoxetine and cocaine induce the epigenetic factors MeCP2 and MBD1 in adult rat brain. Molecular Pharmacology, 70(2), 487–492. doi: 10.1124/mol.106.022301
- Chen, W. G., Chang, Q., Lin, Y., Meissner, A., West, A. E., Griffith, E. C., . . . Greenberg, M. E. (2003). Derepression of BDNF transcription involves calcium-dependent phosphorylation of MeCP2. Science, 302(5646), 885–889.
- Covington, H. E., 3rd, Maze, I., LaPlant, Q. C., Vialou, V. F., Ohnishi, Y. N., Berton, O., . . . Nestler, E. J. (2009). Antidepressant actions of histone deacetylase inhibitors. Journal of Neuroscience, 29(37), 11451–11460. doi: 10.1523/JNEUROSCI.1758-09.2009
- Davie, J. R., & Chadee, D. N. (1998). Regulation and regulatory parameters of histone modifications. *Journal of Cellular Biochemistry Suppl, 30–31 *, 203–213.
- Day, J. J., & Sweatt, J. D. (2011). Epigenetic mechanisms in cognition. Neuron, 70(5), 813–829. doi: 10.1016/j.neuron.2011.05.019
- Dick, D. M., Riley, B., & Kendler, K. S. (2010). Nature and nurture in neuropsychiatric genetics: where do we stand? Dialogues in Clinical Neuroscience, 12(1), 7–23.
- Dolinoy, D. C., Weidman, J. R., & Jirtle, R. L. (2007). Epigenetic gene regulation: linking early developmental environment to adult disease. Reproductive Toxicology, 23(3), 297–307. doi: S0890-6238(06)00197-3 [pii], 10.1016/j.reprotox.2006.08.012
- Feng, J., Chang, H., Li, E., & Fan, G. (2005). Dynamic expression of de novo DNA methyltransferases Dnmt3a and Dnmt3b in the central nervous system. Journal of Neuroscience Research, 79(6), 734–746. doi: 10.1002/jnr.20404
- Feng, J., Zhou, Y., Campbell, S. L., Le, T., Li, E., Sweatt, J. D., . . . Fan, G. (2010). Dnmt1 and Dnmt3a maintain DNA methylation and regulate synaptic function in adult forebrain neurons. Nature Neuroscience, 13(4), 423–430. doi: 10.1038/nn.2514
- Francis, D., Diorio, J., Liu, D., & Meaney, M. J. (1999). Nongenomic transmission across generations of maternal behavior and stress responses in the rat. Science, 286(5442), 1155–1158.
- Gershon, E. S., Alliey-Rodriguez, N., & Liu, C. (2011). After GWAS: searching for genetic risk for schizophrenia and bipolar disorder. American Journal of Psychiatry, 168(3), 253–256. doi: 10.1176/appi.ajp.2010.10091340
- Goll, M. G., & Bestor, T. H. (2005). Eukaryotic cytosine methyltransferases. Annual Review of Biochemistry, 74, 481–514. doi: 10.1146/annurev.biochem.74.010904.153721
- Goto, K., Numata, M., Komura, J. I., Ono, T., Bestor, T. H., & Kondo, H. (1994). Expression of DNA methyltransferase gene in mature and immature neurons as well as proliferating cells in mice. Differentiation, 56(1–2), 39–44.
- Gregg, C., Zhang, J., Weissbourd, B., Luo, S., Schroth, G. P., Haig, D., & Dulac, C. (2010). High-resolution analysis of parent-of-origin allelic expression in the mouse brain. Science, 329(5992), 643–648. doi: 10.1126/science.1190830
- Guan, J. S., Haggarty, S. J., Giacometti, E., Dannenberg, J. H., Joseph, N., Gao, J., . . . Tsai, L. H. (2009). HDAC2 negatively regulates memory formation and synaptic plasticity. Nature, 459(7243), 55-60. doi: 10.1038/nature07925
- Guan, Z., Giustetto, M., Lomvardas, S., Kim, J. H., Miniaci, M. C., Schwartz, J. H., . . . Kandel, E. R. (2002). Integration of long-term-memory-related synaptic plasticity involves bidirectional regulation of gene expression and chromatin structure. Cell, 111(4), 483–493.
- Guo, J. U., Ma, D. K., Mo, H., Ball, M. P., Jang, M. H., Bonaguidi, M. A., . . . Song, H. (2011). Neuronal activity modifies the DNA methylation landscape in the adult brain. Nature Neuroscience, 14(10), 1345–1351. doi: 10.1038/nn.2900
- Heijmans, B. T., Tobi, E. W., Stein, A. D., Putter, H., Blauw, G. J., Susser, E. S., . . . Lumey, L. H. (2008). Persistent epigenetic differences associated with prenatal exposure to famine in humans. Proceedings of the National Academy of Sciences U S A, 105(44), 17046–17049. doi: 0806560105 [pii]10.1073/pnas.0806560105
- Hong, L., Schroth, G. P., Matthews, H. R., Yau, P., & Bradbury, E. M. (1993). Studies of the DNA binding properties of histone H4 amino terminus. Thermal denaturation studies reveal that acetylation markedly reduces the binding constant of the H4 "tail" to DNA. Journal of Biological Chemistry, 268(1), 305–314.
- Jenuwein, T., & Allis, C. D. (2001). Translating the histone code. Science, 293(5532), 1074–1080. doi: 10.1126/Science.1063127293/5532/1074 [pii]
- Jiang, Y. H., Bressler, J., & Beaudet, A. L. (2004). Epigenetics and human disease. Annual Review of Genomics and Human Genetics, 5, 479–510. doi: 10.1146/annurev.genom.5.061903.180014
- Josselyn, S. A. (2005). What's right with my mouse model? New insights into the molecular and cellular basis of cognition from mouse models of Rubinstein-Taybi Syndrome. Learning & Memory, 12(2), 80–83. doi: 12/2/80 [pii]10.1101/lm.93505
- Kadonaga, J. T. (1998). Eukaryotic transcription: an interlaced network of transcription factors and chromatin-modifying machines. Cell, 92(3), 307–313.
- Kalkhoven, E., Roelfsema, J. H., Teunissen, H., den Boer, A., Ariyurek, Y., Zantema, A., . . . Peters, D. J. (2003). Loss of CBP acetyltransferase activity by PHD finger mutations in Rubinstein-Taybi syndrome. Human Molecular Genetics, 12(4), 441–450.
- Korzus, E., Rosenfeld, M. G., & Mayford, M. (2004). CBP histone acetyltransferase activity is a critical component of memory consolidation. Neuron, 42(6), 961–972. doi: 10.1016/j.neuron.2004.06.002S0896627304003526 [pii]
- Kuo, M. H., & Allis, C. D. (1998). Roles of histone acetyltransferases and deacetylases in gene regulation. Bioessays, 20(8), 615–626. doi: 10.1002/(SICI)1521–1878(199808)20:8<615::AID-BIES4>3.0.CO;2-H [pii] 10.1002/(SICI)1521-1878(199808)20:8<615::AID-BIES4>3.0.CO;2-H
- Law, J. A., & Jacobsen, S. E. (2010). Establishing, maintaining and modifying DNA methylation patterns in plants and animals. Nature Reviews Genetics, 11(3), 204–220. doi: nrg2719 [pii]10.1038/nrg2719
- Lee, M. G., Wynder, C., Schmidt, D. M., McCafferty, D. G., & Shiekhattar, R. (2006). Histone H3 lysine 4 demethylation is a target of nonselective antidepressive medications. Chemistry & Biology, 13(6), 563–567. doi: 10.1016/j.chembiol.2006.05.004
- Levenson, J. M., O'Riordan, K. J., Brown, K. D., Trinh, M. A., Molfese, D. L., & Sweatt, J. D. (2004). Regulation of histone acetylation during memory formation in the hippocampus. Journal of Biological Chemistry, 279(39), 40545–40559.
- Li, H., Zhong, X., Chau, K. F., Williams, E. C., & Chang, Q. (2011). Loss of activity-induced phosphorylation of MeCP2 enhances synaptogenesis, LTP and spatial memory. Nature Neuroscience, 14(8), 1001–1008. doi: 10.1038/nn.2866
- Lillycrop, K. A., Phillips, E. S., Jackson, A. A., Hanson, M. A., & Burdge, G. C. (2005). Dietary protein restriction of pregnant rats induces and folic acid supplementation prevents epigenetic modification of hepatic gene expression in the offspring. Journal of Nutrition, 135(6), 1382–1386. doi: 135/6/1382 [pii]
- Lillycrop, K. A., Slater-Jefferies, J. L., Hanson, M. A., Godfrey, K. M., Jackson, A. A., & Burdge, G. C. (2007). Induction of altered epigenetic regulation of the hepatic glucocorticoid receptor in the offspring of rats fed a protein-restricted diet during pregnancy suggests that reduced DNA methyltransferase-1 expression is involved in impaired DNA methylation and changes in histone modifications. British Journal of Nutrition, 97(6), 1064–1073. doi: S000711450769196X [pii]10.1017/S000711450769196X
- Liu, D., Diorio, J., Tannenbaum, B., Caldji, C., Francis, D., Freedman, A., . . . Meaney, M. J. (1997). Maternal care, hippocampal glucocorticoid receptors, and hypothalamic- pituitary-adrenal responses to stress [see comments]. Science, 277(5332), 1659–1662.
- Lumey, L. H., & Stein, A. D. (1997). Offspring birth weights after maternal intrauterine undernutrition: a comparison within sibships. American Journal of Epidemiology, 146(10), 810–819.
- Lutter, M., Krishnan, V., Russo, S. J., Jung, S., McClung, C. A., & Nestler, E. J. (2008). Orexin signaling mediates the antidepressant-like effect of calorie restriction. Journal of Neuroscience, 28(12), 3071–3075. doi: 10.1523/JNEUROSCI.5584-07.2008
- Martinowich, K., Hattori, D., Wu, H., Fouse, S., He, F., Hu, Y., . . . Sun, Y. E. (2003). DNA methylation-related chromatin remodeling in activity-dependent BDNF gene regulation. Science, 302(5646), 890–893.
- McGowan, P. O., Sasaki, A., D'Alessio, A. C., Dymov, S., Labonte, B., Szyf, M., . . . Meaney, M. J. (2009). Epigenetic regulation of the glucocorticoid receptor in human brain associates with childhood abuse. Nature Neuroscience, 12(3), 342–348. doi: nn.2270 [pii]10.1038/nn.2270
- McGowan, P. O., Sasaki, A., Huang, T. C., Unterberger, A., Suderman, M., Ernst, C., . . . Szyf, M. (2008). Promoter-wide hypermethylation of the ribosomal RNA gene promoter in the suicide brain. PLoS ONE, 3(5), e2085. doi: 10.1371/journal.pone.0002085
- Mill, J., Tang, T., Kaminsky, Z., Khare, T., Yazdanpanah, S., Bouchard, L., . . . Petronis, A. (2008). Epigenomic profiling reveals DNA-methylation changes associated with major psychosis. American Journal of Human Genetics, 82(3), 696–711. doi: 10.1016/j.ajhg.2008.01.008
- Miller, C. A., Gavin, C. F., White, J. A., Parrish, R. R., Honasoge, A., Yancey, C. R., . . . Sweatt, J. D. (2010). Cortical DNA methylation maintains remote memory. Nature Neuroscience, 13(6), 664–666. doi: 10.1038/nn.2560
- Myers, M. M., Brunelli, S. A., Shair, H. N., Squire, J. M., & Hofer, M. A. (1989). Relationships between maternal behavior of SHR and WKY dams and adult blood pressures of cross-fostered F1 pups. Developmental Psychobiology, 22(1), 55–67.
- Oberlander, T. F., Weinberg, J., Papsdorf, M., Grunau, R., Misri, S., & Devlin, A. M. (2008). Prenatal exposure to maternal depression, neonatal methylation of human glucocorticoid receptor gene (NR3C1) and infant cortisol stress responses. Epigenetics, 3(2), 97–106. doi: 6034 [pii]
- Ooi, S. K., O'Donnell, A. H., & Bestor, T. H. (2009). Mammalian cytosine methylation at a glance. Journal of Cell Science, 122(Pt 16), 2787–2791. doi: 122/16/2787 [pii]10.1242/jcs.015123
- Painter, R. C., Roseboom, T. J., & Bleker, O. P. (2005). Prenatal exposure to the Dutch famine and disease in later life: an overview. Reproductive Toxicology, 20(3), 345–352. doi: S0890-6238(05)00088-2 [pii]10.1016/j. Reproductive Toxicology.2005.04.005
- Petronis, A. (2010). Epigenetics as a unifying principle in the aetiology of complex traits and diseases. Nature, 465(7299), 721–727. doi: 10.1038/nature09230
- Poulter, M. O., Du, L., Weaver, I. C., Palkovits, M., Faludi, G., Merali, Z., . . . Anisman, H. (2008). GABAA receptor promoter hypermethylation in suicide brain: implications for the involvement of epigenetic processes. Biological Psychiatry, 64(8), 645–652. doi: 10.1016/j.biopsych.2008.05.028
- Rakyan, V. K., Down, T. A., Balding, D. J., & Beck, S. (2011). Epigenome-wide association studies for common human diseases. Nature Reviews Genetics, 12(8), 529–541. doi: 10.1038/nrg3000
- Razin, A. (1998). CpG methylation, chromatin structure and gene silencing-a three-way connection. European Molecular Biology Organization, 17(17), 4905–4908.
- Schaefer, A., Sampath, S. C., Intrator, A., Min, A., Gertler, T. S., Surmeier, D. J., . . . Greengard, P. (2009). Control of cognition and adaptive behavior by the GLP/G9a epigenetic suppressor complex. Neuron, 64(5), 678–691. doi: 10.1016/j.neuron.2009.11.019
- Schroeder, F. A., Lin, C. L., Crusio, W. E., & Akbarian, S. (2007). Antidepressant-like effects of the histone deacetylase inhibitor, sodium butyrate, in the mouse. Biological Psychiatry, 62(1), 55-64. doi: 10.1016/j.biopsych.2006.06.036
- Sealy, L., & Chalkley, R. (1978). DNA associated with hyperacetylated histone is preferentially digested by DNase I. Nucleic Acids Research, 5(6), 1863–1876.
- Shahbazian, M., Young, J., Yuva-Paylor, L., Spencer, C., Antalffy, B., Noebels, J., . . . Zoghbi, H. (2002). Mice with truncated MeCP2 recapitulate many Rett syndrome features and display hyperacetylation of histone H3. Neuron, 35(2), 243–254.
- Skene, P. J., Illingworth, R. S., Webb, S., Kerr, A. R., James, K. D., Turner, D. J., . . . Bird, A. P. (2010). Neuronal MeCP2 is expressed at near histone-octamer levels and globally alters the chromatin state. Molecular Cell, 37(4), 457–468. doi: 10.1016/j.molcel.2010.01.030
- Stanner, S. A., Bulmer, K., Andres, C., Lantseva, O. E., Borodina, V., Poteen, V. V., & Yudkin, J. S. (1997). Does malnutrition in utero determine diabetes and coronary heart disease in adulthood? Results from the Leningrad siege study, a cross sectional study. British Medical Journal, 315(7119), 1342–1348.
- Stern, J. M. (1997). Offspring-induced nurturance: animal-human parallels. Developmental Psychobiololgy, 31(1), 19–37.
- Sutter, D., Doerfler, W., 1980. Methylation of integrated adenovirus type 12 DNA sequences in transformed cells is inversely correlated with viral gene expression. Proceedings of the National Academy of Sciences U S A. 77, 253–256.
- Suzuki, M. M., & Bird, A. (2008). DNA methylation landscapes: provocative insights from epigenomics. Nature Reviews Genetics, 9(6), 465–476. doi: nrg2341 [pii]10.1038/nrg2341
- Tsankova, N. M., Berton, O., Renthal, W., Kumar, A., Neve, R. L., & Nestler, E. J. (2006). Sustained hippocampal chromatin regulation in a mouse model of depression and antidepressant action. Nature Neuroscience. 9(4): 519–525. doi:10.1038/nn1659
- Turner, J. D., Pelascini, L. P., Macedo, J. A., & Muller, C. P. (2008). Highly individual methylation patterns of alternative glucocorticoid receptor promoters suggest individualized epigenetic regulatory mechanisms. Nucleic Acids Research, 36(22), 7207–7218. doi: gkn897 [pii] 10.1093/nar/gkn897
- Vardimon, L., Kressmann, A., Cedar, H., Maechler, M., Doerfler, W., 1982. Expression of a cloned adenovirus gene is inhibited by in vitro methylation. Proceedings of the National Academy of Sciences U S A. 79, 1073–1077.
- Waddington, C. H. (1942). Epigenotype. Endeavour(1), 18–21.
- Wade, P. A., Pruss, D., & Wolffe, A. P. (1997). Histone acetylation: chromatin in action. Trends in Biochemical Sciences, 22(4), 128–132. doi: S0968000497010165 [pii]
- Wang, J., Weaver, I. C., Gauthier-Fisher, A., Wang, H., He, L., Yeomans, J., . . . Miller, F. D. (2010). CBP histone acetyltransferase activity regulates embryonic neural differentiation in the normal and Rubinstein-Taybi syndrome brain. Developmental Cell, 18(1), 114–125. doi: 10.1016/j.devcel.2009.10.023
- Weaver, I. C., Cervoni, N., Champagne, F. A., D'Alessio, A. C., Sharma, S., Seckl, J. R., . . . Meaney, M. J. (2004). Epigenetic programming by maternal behavior. Nature Neuroscience, 7(8), 847–854. doi: 10.1038/nn1276
- Weaver, I. C., Champagne, F. A., Brown, S. E., Dymov, S., Sharma, S., Meaney, M. J., & Szyf, M. (2005). Reversal of maternal programming of stress responses in adult offspring through methyl supplementation: altering epigenetic marking later in life. Journal of Neuroscience, 25(47), 11045–11054. doi: 10.1523/JNEUROSCI.3652-05.2005
- Weaver, I. C., Meaney, M. J., & Szyf, M. (2006). Maternal care effects on the hippocampal transcriptome and anxiety-mediated behaviors in the offspring that are reversible in adulthood. Proceedings of the National Academy of Sciences U S A, 103(9), 3480–3485. doi: 10.1073/pnas.0507526103
- Wells, J. C. (2003). The thrifty phenotype hypothesis: thrifty offspring or thrifty mother? Journal of Theoretical Biology, 221(1), 143–161.
- Wilkinson, M. B., Xiao, G., Kumar, A., LaPlant, Q., Renthal, W., Sikder, D., . . . Nestler, E. J. (2009). Imipramine treatment and resiliency exhibit similar chromatin regulation in the mouse nucleus accumbens in depression models. Journal of Neuroscience, 29(24), 7820–7832. doi: 10.1523/JNEUROSCI.0932-09.2009
- Wolffe, A. P., & Matzke, M. A. (1999). Epigenetics: regulation through repression. Science, 286(5439), 481–486.
How to cite this Chapter using APA Style:
Weaver, I. (2019). Epigenetics in psychology. Adapted for use by Queen's University. Original chapter in R. Biswas-Diener & E. Diener (Eds), Noba textbook series: Psychology.Champaign, IL: DEF publishers. Retrieved from http://noba.to/37p5cb8v
Copyright and Acknowledgment:
This material is licensed under the Creative Commons Attribution-NonCommercial-ShareAlike 4.0 International License. To view a copy of this license, visit: http://creativecommons.org/licenses/by-nc-sa/4.0/deed.en_US.
This material is attributed to the Diener Education Fund (copyright © 2018) and can be accessed via this link: http://noba.to/37p5cb8v.
Additional information about the Diener Education Fund (DEF) can be accessed here.