10 Neurons
Original chapter by Sharon Furtak adapted by the Queen’s University Psychology Department
This Open Access chapter was originally written for the NOBA project. Information on the NOBA project can be found below.
This module on the biological basis of behavior provides an overview of the basic structure of neurons and their means of communication. Neurons, cells in the central nervous system, receive information from our sensory systems (vision, audition, olfaction, gustation, and somatosensation) about the world around us; in turn, they plan and execute appropriate behavioral responses, including attending to a stimulus, learning new information, speaking, eating, mating, and evaluating potential threats. The goal of this module is to become familiar with the anatomical structure of neurons and to understand how neurons communicate by electrochemical signals to process sensory information and produce complex behaviors through networks of neurons. Having a basic knowledge of the fundamental structure and function of neurons is a necessary foundation as you move forward in the field of psychology.
Learning Objectives
- Differentiate the functional roles between the two main cell classes in the brain, neurons and glia.
- Describe how the forces of diffusion and electrostatic pressure work collectively to facilitate electrochemical communication.
- Define resting membrane potential, excitatory postsynaptic potentials, inhibitory postsynaptic potentials, and action potentials.
- Explain features of axonal and synaptic communication in neurons.
Introduction
Imagine trying to string words together into a meaningful sentence without knowing the meaning of each word or its function (i.e., Is it a verb, a noun, or an adjective?). In a similar fashion, to appreciate how groups of cells work together in a meaningful way in the brain as a whole, we must first understand how individual cells in the brain function. Much like words, brain cells, called neurons, have an underlying structure that provides the foundation for their functional purpose. Have you ever seen a neuron? Did you know that the basic structure of a neuron is similar whether it is from the brain of a rat or a human? How do the billions of neurons in our brain allow us to do all the fun things we enjoy, such as texting a friend, cheering on our favorite sports team, or laughing?
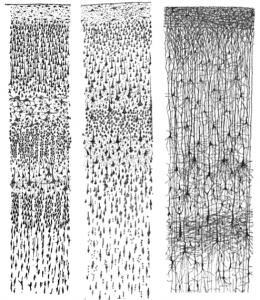
Our journey in answering these questions begins more than 100 years ago with a scientist named Santiago Ramón y Cajal. Ramón y Cajal (1911) boldly concluded that discrete individual neurons are the structural and functional units of the nervous system. He based his conclusion on the numerous drawings he made of Golgi-stained tissue, a stain named after the scientist who discovered it, Camillo Golgi. Scientists use several types of stains to visualize cells. Each stain works in a unique way, which causes them to look differently when viewed under a microscope. For example, a very common Nissl stain labels only the main part of the cell (i.e., the cell body; see left and middle panels of Figure 1). In contrast, a Golgi stain fills the cell body and all the processes that extend outward from it (see right panel of Figure 1). A more notable characteristic of a Golgi stain is that it only stains approximately 1–2% of neurons (Pasternak & Woolsey, 1975; Smit & Colon, 1969), permitting the observer to distinguish one cell from another. These qualities allowed Cajal to examine the full anatomical structure of individual neurons for the first time. This significantly enhanced our appreciation of the intricate networks their processes form. Based on his observation of Golgi-stained tissue, Cajal suggested neurons were distinguishable processing units rather than continuous structures. This was in opposition to the dominant theory at the time proposed by Joseph von Gerlach, which stated that the nervous system was composed of a continuous network of nerves (for review see, Lopez-Munoz, Boya, & Alamo, 2006). Camillo Golgi himself had been an avid supporter of Gerlach’s theory. Despite their scientific disagreement, Cajal and Camillo Golgi shared the Nobel Prize for Medicine in 1906 for their combined contribution to the advancement of science and our understanding of the structure of the nervous system. This seminal work paved the pathway to our current understanding of the basic structure of the nervous system described in this module (for review see: De Carlos & Borrell, 2007; Grant, 2007).
Before moving forward, there will be an introduction to some basic terminology regarding the anatomy of neurons in the section called “The Structure of the Neuron,” below. Once we have reviewed this fundamental framework, the remainder of the module will focus on the electrochemical signals through which neurons communicate. While the electrochemical process might sound intimidating, it will be broken down into digestible sections. The first subsection, “Resting Membrane Potential,” describes what occurs in a neuron at rest, when it is theoretically not receiving or sending signals. Building upon this knowledge, we will examine the electrical conductance that occurs within a single neuron when it receives signals. Finally, the module will conclude with a description of the electrical conductance, which results in communication between neurons through a release of chemicals. At the end of the module, you should have a broad concept of how each cell and large groups of cells send and receive information by electrical and chemical signals.
A note of encouragement: This module introduces a vast amount of technical terminology that at times may feel overwhelming. Do not get discouraged or bogged down in the details. Utilize the glossary at the end of the module as a quick reference guide; tab the glossary page so that you can easily refer to it while reading the module. The glossary contains all terms in bold typing. Terms in italics are additional significant terms that may appear in other modules but are not contained within the glossary. On your first read of this module, I suggest focusing on the broader concepts and functional aspects of the terms instead of trying to commit all the terminology to memory. That is right, I said read first! I highly suggest reading this module at least twice, once prior to and again following the course lecture on this material. Repetition is the best way to gain clarity and commit to memory the challenging concepts and detailed vocabulary presented here.
The Structure of the Neuron
Basic Nomenclature
There are approximately 100 billion neurons in the human brain (Williams & Herrup, 1988). Each neuron has three main components: dendrites, the soma, and the axon (see Figure 2). Dendrites are processes that extend outward from the soma, or cell body, of a neuron and typically branch several times. Dendrites receive information from thousands of other neurons and are the main source of input of the neuron. The nucleus, which is located within the soma, contains genetic information, directs protein synthesis, and supplies the energy and the resources the neuron needs to function. The main source of output of the neuron is the axon. The axon is a process that extends far away from the soma and carries an important signal called an action potential to another neuron. The place at which the axon of one neuron comes in close contact to the dendrite of another neuron is a synapse (see Figures 2–3). Typically, the axon of a neuron is covered with an insulating substance called a myelin sheath that allows the signal and communication of one neuron to travel rapidly to another neuron.
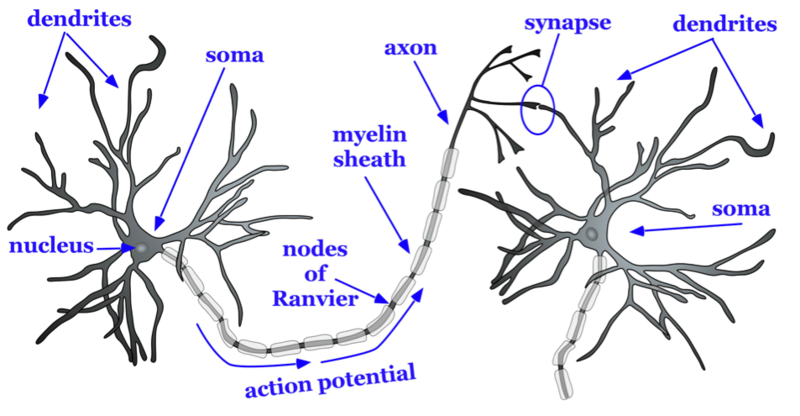
The axon splits many times, so that it can communicate, or synapse, with several other neurons (see Figure 2). At the end of the axon is a terminal button, which forms synapses with spines, or protrusions, on the dendrites of neurons. Synapses form between the presynaptic terminal button (neuron sending the signal) and the postsynaptic membrane (neuron receiving the signal; see Figure 3). Here we will focus specifically on synapses between the terminal button of an axon and a dendritic spine; however, synapses can also form between the terminal button of an axon and the soma or the axon of another neuron.
A very small space called a synaptic gap or a synaptic cleft, approximately 5 nm (nanometers), exists between the presynaptic terminal button and the postsynaptic dendritic spine. To give you a better idea of the size, a dime is 1.35 mm (millimeter) thick. There are 1,350,000 nm in the thickness of a dime. In the presynaptic terminal button, there are synaptic vesicles that package together groups of chemicals called neurotransmitters (see Figure 3). Neurotransmitters are released from the presynaptic terminal button, travel across the synaptic gap, and activate ion channels on the postsynaptic spine by binding to receptor sites. We will discuss the role of receptors in more detail later in the module.
Types of Cells in the Brain
Not all neurons are created equal! There are neurons that help us receive information about the world around us, sensory neurons. There are motor neurons that allow us to initiate movement and behavior, ultimately allowing us to interact with the world around us. Finally, there are interneurons, which process the sensory input from our environment into meaningful representations, plan the appropriate behavioral response, and connect to the motor neurons to execute these behavioral plans.
There are three main categories of neurons, each defined by its specific structure. The structures of these three different types of neurons support their unique functions. Unipolar neurons are structured in such a way that is ideal for relaying information forward, so they have one neurite (axon) and no dendrites. They are involved in transmission of physiological information from the body’s periphery such as communicating body temperature through the spinal cord up to the brain. Bipolar neurons are involved in sensory perception such as perception of light in the retina of the eye. They have one axon and one dendrite which help acquire and pass sensory information to various centers in the brain. Finally, multipolar neurons are the most common and they communicate sensory and motor information in the brain. For example, their firing causes muscles in the body to contract. Multipolar neurons have one axon and many dendrites which allows them to communicate with other neurons. One of the most prominent neurons is a pyramidal neuron, which falls under the multipolar category. It gets its name from the triangular or pyramidal shape of its soma (for examples see, Furtak, Moyer, & Brown, 2007).
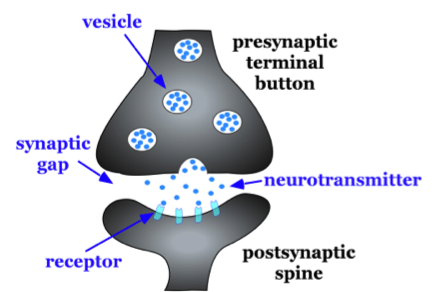
In addition to neurons, there is a second type of cell in the brain called glia cells. Glia cells have several functions, just a few of which we will discuss here. One type of glia cell, called oligodendroglia, forms the myelin sheaths mentioned above (Simons & Trotter, 2007; see Fig. 2). Oligodendroglia wrap their dendritic processes around the axons of neurons many times to form the myelin sheath. One cell will form the myelin sheath on several axons. Other types of glia cells, such as microglia and astrocytes, digest debris of dead neurons, carry nutritional support from blood vessels to the neurons, and help to regulate the ionic composition of the extracellular fluid. While glial cells play a vital role in neuronal support, they do not participate in the communication between cells in the same fashion as neurons do.
Communication Within and Between Neurons
Thus far, we have described the main characteristics of neurons, including how their processes come in close contact with one another to form synapses. In this section, we consider the conduction of communication within a neuron and how this signal is transmitted to the next neuron. There are two stages of this electrochemical action in neurons. The first stage is the electrical conduction of dendritic input to the initiation of an action potential within a neuron. The second stage is a chemical transmission across the synaptic gap between the presynaptic neuron and the postsynaptic neuron of the synapse. To understand these processes, we first need to consider what occurs within a neuron when it is at a steady state, called resting membrane potential.
Resting Membrane Potential
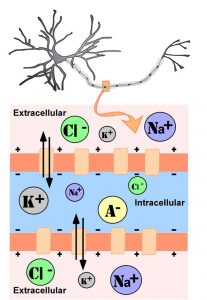
The intracellular (inside the cell) fluid and extracellular (outside the cell) fluid of neurons is composed of a combination of ions (electrically charged molecules; see Figure 4). Cations are positively charged ions, and anions are negatively charged ions. The composition of intracellular and extracellular fluid is similar to salt water, containing sodium (Na+), potassium (K+), chloride (Cl–), and anions (A–).
The cell membrane, which is composed of a lipid bilayer of fat molecules, separates the cell from the surrounding extracellular fluid. There are proteins that span the membrane, forming ion channels that allow particular ions to pass between the intracellular and extracellular fluid (see Figure 4). These ions are in different concentrations inside the cell relative to outside the cell, and the ions have different electrical charges. Due to this difference in concentration and charge, two forces act to maintain a steady state when the cell is at rest: diffusion and electrostatic pressure. Diffusion is the force on molecules to move from areas of high concentration to areas of low concentration. Electrostatic pressure is the force on two ions with similar charge to repel each other and the force of two ions with opposite charge to attract to one another. Remember the saying, opposites attract?
Regardless of the ion, there exists a membrane potential at which the force of diffusion is equal and opposite of the force of electrostatic pressure. This voltage, called the equilibrium potential, is the voltage at which no ions flow. Since there are several ions that can permeate the cell’s membrane, the baseline electrical charge inside the cell compared with outside the cell, referred to as resting membrane potential, is based on the collective drive of force on several ions. Relative to the extracellular fluid, the membrane potential of a neuron at rest is negatively charged at approximately -70 mV (see Figure 5). These are very small voltages compared with the voltages of batteries and electrical outlets, which we encounter daily, that range from 1.5 to 240 V.
Let us see how these two forces, diffusion and electrostatic pressure, act on the four groups of ions mentioned above.
- Anions (A-): Anions are highly concentrated inside the cell and contribute to the negative charge of the resting membrane potential. Diffusion and electrostatic pressure are not forces that determine A– concentration because A– is impermeable to the cell membrane. There are no ion channels that allow for A– to move between the intracellular and extracellular fluid.
- Potassium (K+): The cell membrane is very permeable to potassium at rest, but potassium remains in high concentrations inside the cell. Diffusion pushes K+ outside the cell because it is in high concentration inside the cell. However, electrostatic pressure pushes K+ inside the cell because the positive charge of K+ is attracted to the negative charge inside the cell. In combination, these forces oppose one another with respect to K+.
- Chloride (Cl-): The cell membrane is also very permeable to chloride at rest, but chloride remains in high concentration outside the cell. Diffusion pushes Cl– inside the cell because it is in high concentration outside the cell. However, electrostatic pressure pushes Cl– outside the cell because the negative charge of Cl– is attracted to the positive charge outside the cell. Similar to K+, these forces oppose one another with respect to Cl–.
- Sodium (Na+): The cell membrane is not very permeable to sodium at rest. Diffusion pushes Na+ inside the cell because it is in high concentration outside the cell. Electrostatic pressure also pushes Na+ inside the cell because the positive charge of Na+ is attracted to the negative charge inside the cell. Both of these forces push Na+ inside the cell; however, Na+ cannot permeate the cell membrane and remains in high concentration outside the cell. The small amounts of Na+ inside the cell are removed by a sodium-potassium pump, which uses the neuron’s energy (adenosine triphosphate, ATP) to pump 3 Na+ ions out of the cell in exchange for bringing 2 K+ ions inside the cell.
Action Potential
Now that we have considered what occurs in a neuron at rest, let us consider what changes occur to the resting membrane potential when a neuron receives input, or information, from the presynaptic terminal button of another neuron. Our understanding of the electrical signals or potentials that occurs within a neuron results from the seminal work of Hodgkin and Huxleythat began in the 1930s at a well-known marine biology lab in Woodshole, MA. Their work, for which they won the Nobel Prize in Medicine in 1963, has resulted in the general model of electrochemical transduction that is described here (Hodgkin & Huxley, 1952). Hodgkin and Huxley studied a very large axon in the squid, a common species for that region of the United States. The giant axon of the squid is roughly 100 times larger than that of axons in the mammalian brain, making it much easier to see. Activation of the giant axon is responsible for a withdrawal response the squid uses when trying to escape from a predator, such as large fish, birds, sharks, and even humans. When was the last time you had calamari? The large axon size is no mistake in nature’s design; it allows for very rapid transmission of an electrical signal, enabling a swift escape motion in the squid from its predators.
While studying this species, Hodgkin and Huxley noticed that if they applied an electrical stimulus to the axon, a large, transient electrical current conducted down the axon. This transient electrical current is known as an action potential (see Figure 5). An action potential is an all-or-nothing response that occurs when there is a change in the charge or potential of the cell from its resting membrane potential (-70 mV) in a more positive direction, which is a depolarization (see Figure 5). What is meant by an all-or-nothing response? I find that this concept is best compared to the binary code used in computers, where there are only two possibilities, 0 or 1. There is no halfway or in-between these possible values; for example, 0.5 does not exist in binary code. There are only two possibilities, either the value of 0 or the value of 1. The action potential is the same in this respect. There is no halfway; it occurs, or it does not occur. There is a specific membrane potential that the neuron must reach to initiate an action potential. This membrane potential, called the threshold of excitation, is typically around -50 mV. If the threshold of excitation is reached, then an action potential is triggered.
How is an action potential initiated? At any one time, each neuron is receiving hundreds of inputs from the cells that synapse with it. These inputs can cause several types of fluctuations in the neuron’s membrane potentials (see Figure 5):
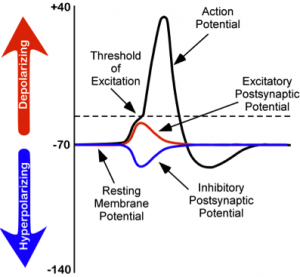
- excitatory postsynaptic potentials (EPSPs): a depolarizing current that causes the membrane potential to become more positive and closer to the threshold of excitation; or
- inhibitory postsynaptic potentials (IPSPs): a hyperpolarizing current that causes the membrane potential to become more negative and further away from the threshold of excitation.
These postsynaptic potentials, EPSPs and IPSPs, summate or add together in time and space. The IPSPs make the membrane potential more negative, but how much so depends on the strength of the IPSPs. The EPSPs make the membrane potential more positive; again, how much more positive depends on the strength of the EPSPs. If you have two small EPSPs at the same time and the same synapse then the result will be a large EPSP. If you have a small EPSP and a small IPSP at the same time and the same synapse then they will cancel each other out. Unlike the action potential, which is an all-or-nothing response, IPSPs and EPSPs are smaller and graded potentials, varying in strength. The change in voltage during an action potential is approximately 100 mV. In comparison, EPSPs and IPSPs are changes in voltage between 0.1 to 40 mV. They can be different strengths, or gradients, and they are measured by how far the membrane potentials diverge from the resting membrane potential.
I know the concept of summation can be confusing. As a child, I use to play a game in elementary school with a very large parachute where you would try to knock balls out of the center of the parachute. This game illustrates the properties of summation rather well. In this game, a group of children next to one another would work in unison to produce waves in the parachute in order to cause a wave large enough to knock the ball out of the parachute. The children would initiate the waves at the same time and in the same direction. The additive result was a larger wave in the parachute, and the balls would bounce out of the parachute. However, if the waves they initiated occurred in the opposite direction or with the wrong timing, the waves would cancel each other out, and the balls would remain in the center of the parachute. EPSPs or IPSPs in a neuron work in the same fashion to the properties of the waves in the parachute; they either add or cancel each other out. If you have two EPSPs, then they sum together and become a larger depolarization. Similarly, if two IPSPs come into the cell at the same time, they will sum and become a larger hyperpolarization in membrane potential. However, if two inputs were opposing one another, moving the potential in opposite directions, such as an EPSP and an IPSP, their sum would cancel each other out.
At any moment in time, each cell is receiving mixed messages, both EPSPs and IPSPs. If the summation of EPSPs is strong enough to depolarize the membrane potential to reach the threshold of excitation, then it initiates an action potential. The action potential then travels down the axon, away from the soma, until it reaches the ends of the axon (the terminal button). In the terminal button, the action potential triggers the release of neurotransmitters from the presynaptic terminal button into the synaptic gap. These neurotransmitters, in turn, cause EPSPs and IPSPs in the postsynaptic dendritic spines of the next cell (see Figures 4 & 6). The neurotransmitter released from the presynaptic terminal button binds with ionotropic receptors in a lock-and-key fashion on the post-synaptic dendritic spine. Ionotropic receptors are receptors on ion channels that open, allowing some ions to enter or exit the cell, depending upon the presence of a particular neurotransmitter. The type of neurotransmitter and the permeability of the ion channel it activates will determine if an EPSP or IPSP occurs in the dendrite of the post-synaptic cell. These EPSPs and IPSPs summate in the same fashion described above and the entire process occurs again in another cell.
The Change in Membrane Potential During an Action Potential
We discussed previously which ions are involved in maintaining the resting membrane potential. Not surprisingly, some of these same ions are involved in the action potential. When the cell becomes depolarized (more positively charged) and reaches the threshold of excitation, this causes a voltage-dependent Na+ channel to open. A voltage-dependent ion channel is a channel that opens, allowing some ions to enter or exit the cell, depending upon when the cell reaches a particular membrane potential. When the cell is at resting membrane potential, these voltage-dependent Na+ channels are closed. As we learned earlier, both diffusion and electrostatic pressure are pushing Na+ inside the cells. However, Na+ cannot permeate the membrane when the cell is at rest. Now that these channels are open, Na+ rushes inside the cell, causing the cell to become very positively charged relative to the outside of the cell. This is responsible for the rising or depolarizing phase of the action potential (see Figure 5). The inside of the cell becomes very positively charged, +40mV. At this point, the Na+ channels close and become refractory. This means the Na+ channels cannot reopen again until after the cell returns to the resting membrane potential. Thus, a new action potential cannot occur during the refractory period. The refractory period also ensures the action potential can only move in one direction down the axon, away from the soma. As the cell becomes more depolarized, a second type of voltage-dependent channel opens; this channel is permeable to K+. With the cell very positive relative to the outside of the cell (depolarized) and the high concentration of K+ within the cell, both the force of diffusion and the force of electrostatic pressure drive K+ outside of the cell. The movement of K+ out of the cell causes the cell potential to return back to the resting membrane potential, the falling or hyperpolarizing phase of the action potential (see Figure 5). A short hyperpolarization occurs partially due to the gradual closing of the K+ channels. With the Na+ closed, electrostatic pressure continues to push K+ out of the cell. In addition, the sodium-potassium pump is pushing Na+ out of the cell. The cell returns to the resting membrane potential, and the excess extracellular K+ diffuses away. This exchange of Na+ and K+ ions happens very rapidly, in less than 1 msec. The action potential occurs in a wave-like motion down the axon until it reaches the terminal button. Only the ion channels in very close proximity to the action potential are affected.
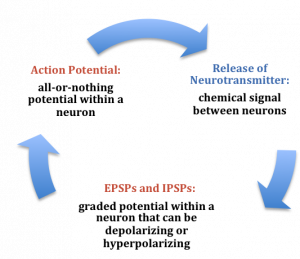
Earlier you learned that axons are covered in myelin. Let us consider how myelin speeds up the process of the action potential. There are gaps in the myelin sheaths called nodes of Ranvier. The myelin insulates the axon and does not allow any fluid to exist between the myelin and cell membrane. Under the myelin, when the Na+ and K+ channels open, no ions flow between the intracellular and extracellular fluid. This saves the cell from having to expend the energy necessary to rectify or regain the resting membrane potential. (Remember, the pumps need ATP to run.) Under the myelin, the action potential degrades some, but is still large enough in potential to trigger a new action potential at the next node of Ranvier. Thus, the action potential actively jumps from node to node; this process is known as saltatory conduction.
In the presynaptic terminal button, the action potential triggers the release of neurotransmitters (see Figure 3). Neurotransmitters cross the synaptic gap and open subtypes of receptors in a lock-and-key fashion (see Figure 3). Depending on the type of neurotransmitter, an EPSP or IPSP occurs in the dendrite of the post-synaptic cell. Neurotransmitters that open Na+ or calcium (Ca+) channels cause an EPSP; an example is the NMDA receptors, which are activated by glutamate (the main excitatory neurotransmitter in the brain). In contrast, neurotransmitters that open Cl- or K+ channels cause an IPSP; an example is gamma-aminobutryric acid (GABA) receptors, which are activated by GABA, the main inhibitory neurotransmitter in the brain. Once the EPSPs and IPSPs occur in the postsynaptic site, the process of communication within and between neurons cycles on (see Figure 6). A neurotransmitter that does not bind to receptors is broken down and inactivated by enzymes or glial cells, or it is taken back into the presynaptic terminal button in a process called reuptake, which will be discussed further in the module on psychopharmacology.
Check Your Knowledge
To help you with your studying, we’ve included some practice questions for this module. These questions do not necessarily address all content in this module. They are intended as practice, and you are responsible for all of the content in this module even if there is no associated practice question. To promote deeper engagement with the material, we encourage you to create some questions of your own for your practice. You can then also return to these self-generated questions later in the course to test yourself.
Vocabulary
- Action potential
- A transient all-or-nothing electrical current that is conducted down the axon when the membrane potential reaches the threshold of excitation.
- Axon
- Part of the neuron that extends off the soma, splitting several times to connect with other neurons; main output of the neuron.
- Cell membrane
- A bi-lipid layer of molecules that separates the cell from the surrounding extracellular fluid.
- Dendrite
- Part of a neuron that extends away from the cell body and is the main input to the neuron.
- Diffusion
- The force on molecules to move from areas of high concentration to areas of low concentration.
- Electrostatic pressure
- The force on two ions with similar charge to repel each other; the force of two ions with opposite charge to attract to one another.
- Excitatory postsynaptic potentials
- A depolarizing postsynaptic current that causes the membrane potential to become more positive and move towards the threshold of excitation.
- Inhibitory postsynaptic potentials
- A hyperpolarizing postsynaptic current that causes the membrane potential to become more negative and move away from the threshold of excitation.
- Ion channels
- Proteins that span the cell membrane, forming channels that specific ions can flow through between the intracellular and extracellular space.
- Ionotropic receptor
- Ion channel that opens to allow ions to permeate the cell membrane under specific conditions, such as the presence of a neurotransmitter or a specific membrane potential.
- Myelin sheath
- Substance around the axon of a neuron that serves as insulation to allow the action potential to conduct rapidly toward the terminal buttons.
- Neurotransmitters
- Chemical substance released by the presynaptic terminal button that acts on the postsynaptic cell.
- Nucleus
- Collection of nerve cells found in the brain which typically serve a specific function.
- Resting membrane potential
- The voltage inside the cell relative to the voltage outside the cell while the cell is at rest (approximately -70 mV).
- Sodium-potassium pump
- An ion channel that uses the neuron’s energy (adenosine triphosphate, ATP) to pump three Na+ ions outside the cell in exchange for bringing two K+ ions inside the cell.
- Soma
- Cell body of a neuron that contains the nucleus and genetic information, and directs protein synthesis.
- Spines
- Protrusions on the dendrite of a neuron that form synapses with terminal buttons of the presynaptic axon.
- Synapse
- Junction between the presynaptic terminal button of one neuron and the dendrite, axon, or soma of another postsynaptic neuron.
- Synaptic gap
- Also known as the synaptic cleft; the small space between the presynaptic terminal button and the postsynaptic dendritic spine, axon, or soma.
- Synaptic vesicles
- Groups of neurotransmitters packaged together and located within the terminal button.
- Terminal button
- The part of the end of the axon that form synapses with postsynaptic dendrite, axon, or soma.
- Threshold of excitation
- Specific membrane potential that the neuron must reach to initiate an action potential.
References
- De Carlos, J. A., & Borrell, J. (2007). A historical reflection of the contributions of Cajal and Golgi to the foundations of neuroscience. Brain Res Rev, 55(1), 8-16. doi: 10.1016/j.brainresrev.2007.03.010
- Furtak, S. C., Moyer, J. R., Jr., & Brown, T. H. (2007). Morphology and ontogeny of rat perirhinal cortical neurons. J Comp Neurol, 505(5), 493-510. doi: 10.1002/cne.21516
- Grant, G. (2007). How the 1906 Nobel Prize in Physiology or Medicine was shared between Golgi and Cajal. Brain Res Rev, 55(2), 490-498. doi: 10.1016/j.brainresrev.2006.11.004
- Hodgkin, A. L., & Huxley, A. F. (1952). A quantitative description of membrane current and its application to conduction and excitation in nerve. J Physiol, 117(4), 500-544.
- Lopez-Munoz, F., Boya, J., & Alamo, C. (2006). Neuron theory, the cornerstone of neuroscience, on the centenary of the Nobel Prize award to Santiago Ramon y Cajal. Brain Res Bull, 70(4-6), 391-405. doi: 10.1016/j.brainresbull.2006.07.010
- Pasternak, J. F., & Woolsey, T. A. (1975). On the “selectivity” of the Golgi-Cox method. J Comp Neurol, 160(3), 307-312. doi: 10.1002/cne.901600304
- Ramón y Cajal, S. (1911). Histology of the nervous system of man and vertebrates. New York, NY: Oxford University Press.
- Simons, M., & Trotter, J. (2007). Wrapping it up: the cell biology of myelination. Curr Opin Neurobiol, 17(5), 533-540. doi: 10.1016/j.conb.2007.08.003
- Smit, G. J., & Colon, E. J. (1969). Quantitative analysis of the cerebral cortex. I. Aselectivity of the Golgi-Cox staining technique. Brain Res, 13(3), 485-510.
- Williams, R. W., & Herrup, K. (1988). The control of neuron number. Annu Rev Neurosci, 11, 423-453. doi: 10.1146/annurev.ne.11.030188.002231
How to cite this Chapter using APA Style:
Furtak, S. (2019). Neurons. Adapted for use by Queen’s University. Original chapter in R. Biswas-Diener & E. Diener (Eds), Noba textbook series: Psychology. Champaign, IL: DEF publishers. Retrieved from http://noba.to/s678why4
Copyright and Acknowledgment:
This material is licensed under the Creative Commons Attribution-NonCommercial-ShareAlike 4.0 International License. To view a copy of this license, visit: http://creativecommons.org/licenses/by-nc-sa/4.0/deed.en_US.
This material is attributed to the Diener Education Fund (copyright © 2018) and can be accessed via this link: http://noba.to/s678why4.
Additional information about the Diener Education Fund (DEF) can be accessed here.