4 Drugs for Treatment of Diabetes Mellitus
4.1 Pathology
Diabetes mellitus is a condition that occurs when there is an elevated concentration of glucose in the blood. Glucose is the principal type of carbohydrate that the body uses for energy, and carbohydrates are one of the three macronutrients (along with proteins and lipids). In contrast to hyperlipidemia where there is a high concentration of hydrophobic lipids in the blood, in diabetes mellitus the situation is somewhat reversed. There is a high concentration of sugars in the blood, which are highly polar due to the multiple hydroxyl groups on each sugar molecule. This can lead to the blood becoming very ‘sticky’ and viscous. This can become more serious in organs with tiny blood vessels such as the eyes, kidneys and other extremities as it becomes challenging for the blood to reach these locations and deliver necessary components or immune cells. Therefore, it is very important to maintain appropriate concentrations of blood sugar.
Diabetes mellitus is a chronic condition and there are three different types: Type 1, 2, and gestational diabetes. Type 1 diabetes is generally thought of as a genetic condition, with early onset and lower frequency (~10% of diabetes cases). Type 2 diabetes may also have a genetic component but is largely driven by lifestyle and has a late onset. Gestational diabetes can occur when the patient is pregnant, and usually resolves naturally following the pregnancy term.
The incidence of diabetes in Canada has been increasing over the past two decades (4.7% of the population in 2001, compared to 8.1% in 2017), which may be attributed to changes in diet and lifestyle. Carbohydrates represent the most accessible form of energy for body, and form a large part of the natural human diet. They are digested and absorbed by different organs of the body, which provides a foundation for medicinal chemistry efforts to treat diabetes.
4.1.1 Carbohydrate Digestion – Mouth and Stomach
Carbohydrate digestion begins in the mouth. Although the mechanical action of chewing helps to physically separate carbohydrates, the enzyme salivary amylase starts hydrolyzing some of the complex sugars. Approximately 5% of carbohydrate digestion occurs in the mouth. These enzymes are inactivated by the gastric acid of the stomach. The mechanical action of the stomach coupled to the acidic conditions may help to further separate carbohydrates, but these are not the predominant sites of carbohydrate digestion.
4.1.2 Carbohydrate Digestion – Small Intestine
The stomach empties the mixture of food (now called chyme) into the small intestine, where several enzymes become involved. There is a different type of amylase (pancreatic amylase) that continues breaking down larger sugars. Another enzyme, α-glucosidase, breaks down polymer sugar molecules into monomers, such as glucose or fructose. These monomers can now be absorbed by different transporters. Proteins in cells of the small intestine are expressed asymmetrically on the cell membrane. (Figure 4.1) For example, SGLT1 (sodium glucose transporter) enables glucose transport into the cells from the small intestine lumen. GLUT2 (glucose transporter) faces the blood vessel lumen and deposits glucose in the blood stream. Both of these transporters are important and part of larger families of carbohydrate transporters and have different tissue expression throughout the body. SGLT1 is one of twelve members of the SLC5A (solute carrier family member 1) proteins. GLUT transporters represent a family of fourteen transporters with different affinities for glucose or other carbohydrate monomers (for example GLUT5 absorbs fructose).
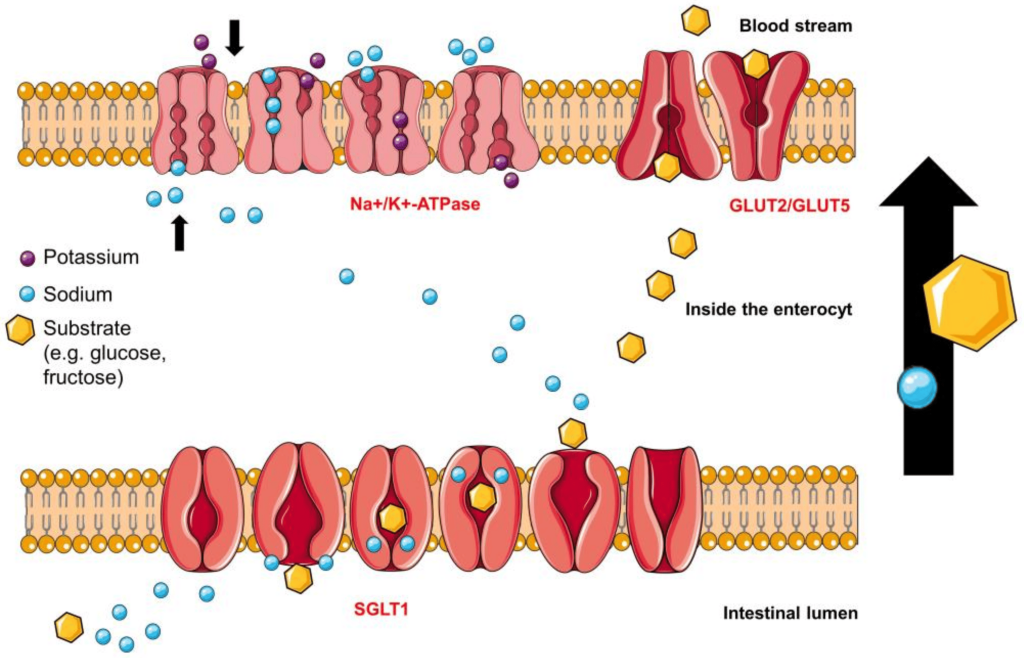
As soon as chyme is released into the small intestine, it also triggers the release of hormones called incretins. (Figure 4.2) There are two incretins that are very important called glucagon-like peptide 1 (GLP1) and glucose-dependent insulinotropic peptide (GIP1). These are polypeptides that are secreted by specific cells of the upper and lower intestine that travel to the pancreas and help prepare it for the incoming spike in blood sugar. In this way, the action of incretins occurs even before any glucose enters the blood stream.
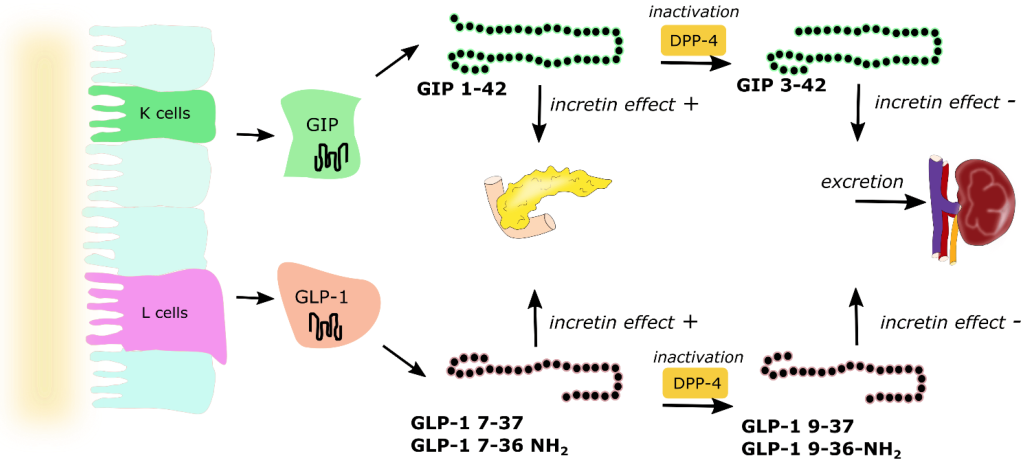
GIP has multiple roles including promoting insulin secretion, suppressing glucagon release, and retarding gastric emptying to provide additional time for digestion thereby softening large peaks in glucose levels. Incretins also provide a feeling of satiety, which reduces food consumption. Incretins are rapidly deactivated by a set of enzymes called DDP-4 (dipeptidyl peptidase) in the blood stream and incretins are only active for a few minutes.
4.1.3 Carbohydrate Digestion – Pancreas
The pancreas is one of the most important organs for regulating blood glucose levels. (Figure 4.3) Once glucose enters the blood stream via the small intestine, multiple organs within the body start to respond. The pancreatic cells harbour GLUT2 transporters (similar to the small intestine) and these transporters uptake the glucose from the blood (specifically the pancreatic β-cells). The increased of intracellular glucose concentrations triggers ATP generation (via glycolysis, Krebs/TCA cycle, etc.) which leads to a high [ATP] state. ATP binds to KATP channels (ATP sensitive potassium channels) which causes the potassium channels to close, leading to a change in membrane polarization. This change triggers opening of voltage gated Ca2+ channels. The increase in Ca2+ ion content triggers secretion of insulin from the β-cells into the blood stream. Insulin is a critical hormone in carbohydrate digestion, and is responsible for making cells more permeable to glucose, which ultimately reduces glucose concentrations in the blood.
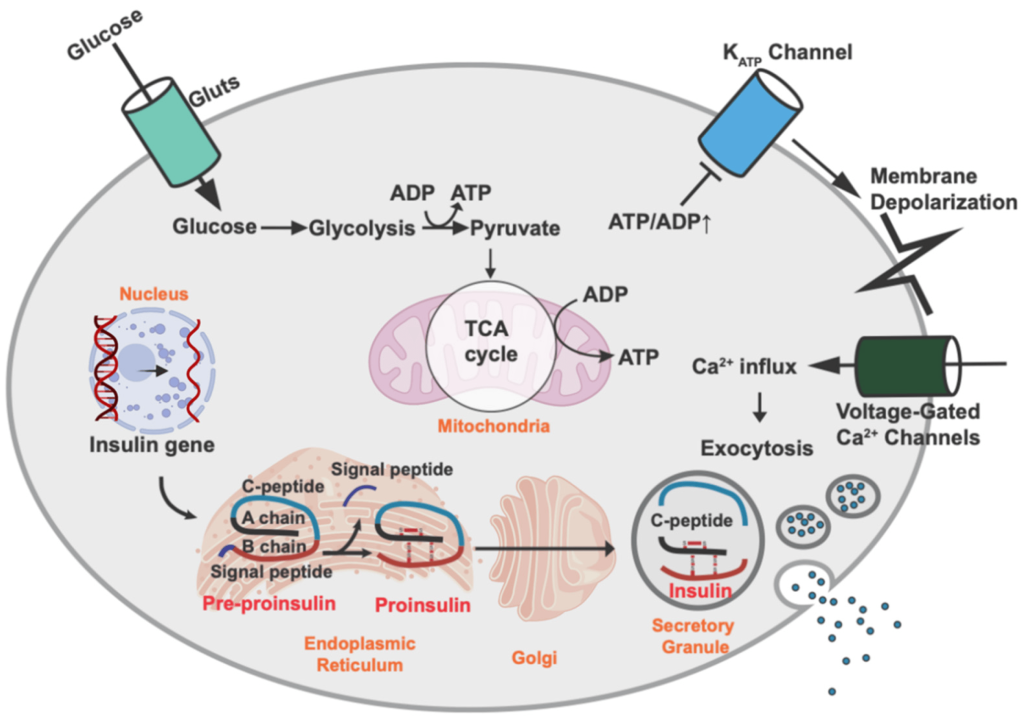
Insulin will travel through the blood stream and bind to insulin receptors on different types of cells. The binding event initiates a signal cascade inside the cell with a net result of glucose transporters translocating to the cell surface. The glucose transporters on skeletal and cardiac muscle are insulin expression dependent GLUT4 (whereas the transporters in the pancreas and small intestine are insulin independent GLUT2 proteins). The presence of these transporters enables the uptake of glucose from the blood providing an energy source to the cells and restoring normal glucose levels.
In cases where energy is required but food intake is not occurring, blood sugar levels can start to fall. The α-cells of the pancreas sense lowering of blood sugar levels and release the polypeptide, glucagon. Glucagon is the complementary hormone to insulin, and it signals the liver to release glucose (which is stored in the liver in a polymer called glycogen). The release of glucose will correspondingly raise blood sugar levels, and in this way, the interplay between the pancreas and liver by way of the hormones, insulin and glucagon, regulates homeostasis in the body. (Figure 4.4)
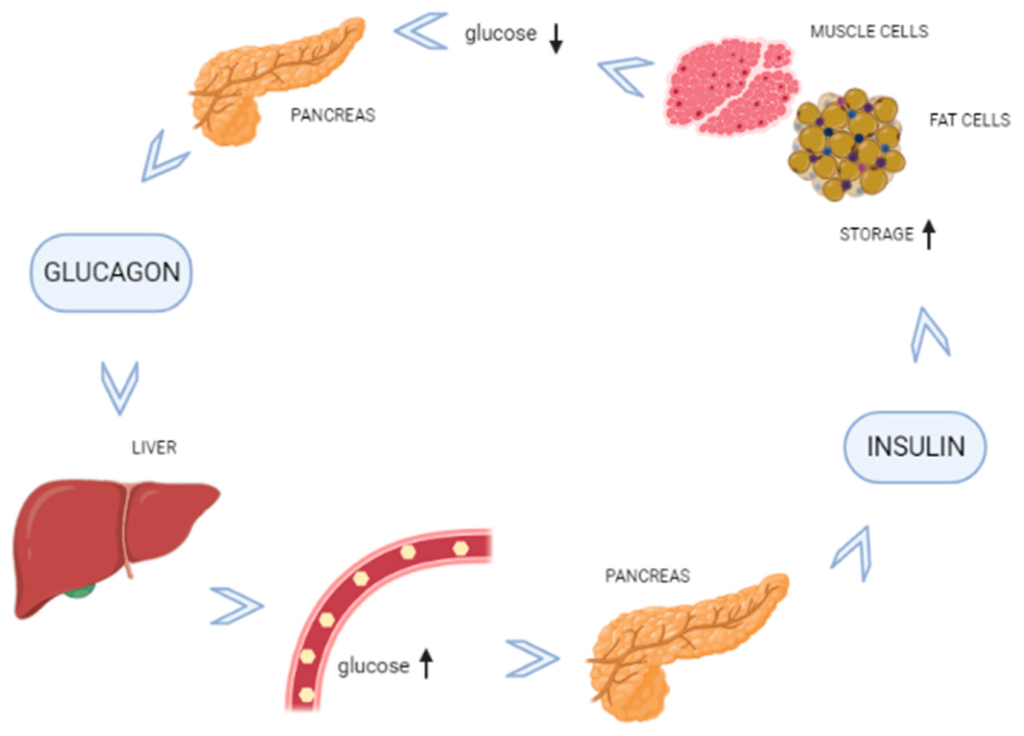
4.1.4 Carbohydrate Digestion – Liver
The liver is critical in maintaining blood sugar levels. The liver stores excess glucose in the form of glycogen (a massive polymer of glucose). The pancreas and liver work in lockstep to ensure blood glucose levels remain constant throughout the daily demands and resources available to the body. Therefore, when the body has extra glucose, the pancreas releases insulin which causes the liver to starting storing glucose, and during periods where blood glucose levels fall, the pancreas releases glucagon which causes the liver to start breaking down glycogen to release glucose in the blood stream.
4.2 Insulin
Insulin is a polypeptide that is naturally synthesized by the pancreas. Insulin is comprised of two polypeptide chains called Chain A (21 amino acids) and Chain B (30 amino acids) with a combined molecular weight of ~6 kDa. Additionally, there are three critical di-sulfide linkages within insulin:
- Intramolecular: Cys6 (Chain A) and Cys11 (Chain A)
- Intermolecular: Cys7 (Chain A) with Cys7 (Chain B)
- Intermolecular: Cys20 (Chain A) and Cys19 (Chain B)
Insulin is biosynthesized as a single polypeptide chain in the β-cells of the pancreas. The ribosomes generate the polypeptide with four continuous segments which is called preproinsulin. (Figure 4.5) The N-terminal segment contains the signal sequence for the excretory pathway, and it is cleaved from the main protein segment in the rough endoplasmic reticulum (the polypeptide is now called proinsulin). This enables the protein to fold over on itself and form the three characteristic di-sulfide bridges. In the trans-Golgi network, the proinsulin peptide is cleaved at two sites to give the final insulin molecule. This is the version of the protein that is excreted by the pancreas beta cells and travels through the blood stream.
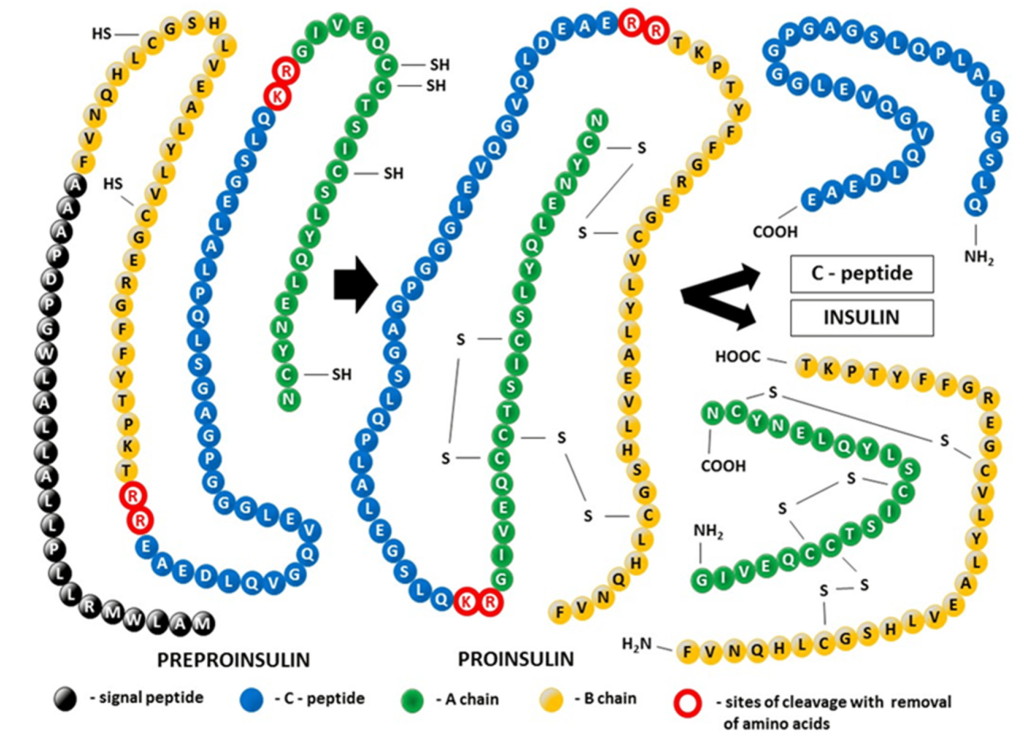
Insulin biosynthesis occurs in the pancreas, and results in very high intracellular concentrations. β-Cells also maintain a relatively high concentration of Zn2+ ions and, coupled to the high concentration of insulin, this causes the insulin peptides to crystallize around the Zn2+ ions. The crystals exhibit a 3-fold symmetry. There are two Zn2+ ions at the centre of the polymer along with 6 insulin molecules surrounding these ions (i.e. a trimer of dimers). Specific histidine residues from chain B on insulin coordinate the Zn2+ ions. (Figure 4.6)
FIGURE 4.6 Insulin monomers combine to form insulin hexamers that coordinate a central zinc ion. Image Source: (Fig 1) by Beatrice Rosetti and Silvia Marchesan is used under a CC-BY 4.0 license & (Fig 2) by Harish Vashisth has been modified (cropped) and is used under a CC-BY 4.0 license.
These hexamers become extremely important pharmacologically. In particular, the insulin hexamers are inactive and cannot perform their natural biological function in this state. However, when the pancreas releases insulin into the blood stream, the concentration of Zn2+ is substantially diluted, and the hexamers rapidly dissociate into monomers and become active. Therefore, the equilibrium and transition from insulin hexamers to monomers is critical. The cellular vessels that store insulin are also quite acidic which further reduces the solubility and increases the crystalline packing of insulin. Insulin has a very short half-life in the blood (~3-10 min) and is rapidly degraded. Therefore, the effects of insulin are short-lived but extremely potent.
4.3 Treatments for Type I Diabetes
Type I diabetes patients may have genetic limitations in producing sufficient quantities of insulin, which means that they will not be able to respond to changes in blood glucose concentrations. One treatment strategy that has emerged is to supply exogenous insulin to the body (which can be prepared synthetically or obtained from biological sources). In this way, the insulin producing functions of β-cells are replaced. (Figure 4.7)
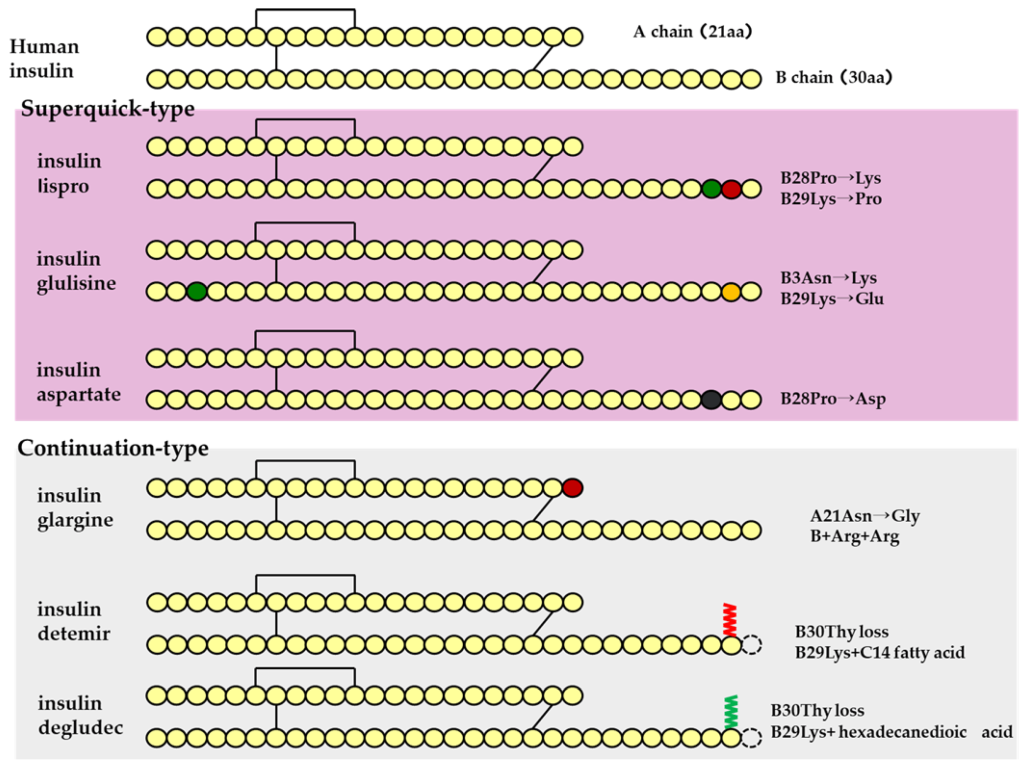
4.3.1 Short Acting Agents
The first strategy involves supplying insulin directly (in the form that it naturally exists in the body). This agent is called Regular Insulin. Regular Insulin has the exact same protein sequence as human insulin, and therefore also forms the same hexameric structure in the presence of Zn2+. The main difference is that Regular Insulin is injected subcutaneously (unlike natural insulin which enters the blood stream directly from pancreatic β-cells). The direct release of natural insulin into the blood stream leads to near instantaneous dissociation of the hexamers. However, in subcutaneous injections, the hexamers need to dissociate into smaller monomers before they can find their way into the blood stream. This means that there is a delayed onset of action, as the insulin needs to dissociate into monomers before it can be effectively absorbed, which can take approximately 30 – 60 min. This can be inconvenient, because if a patient is consuming food, they would need to administer an injection and wait for ~30 min before eating.
4.3.2 Rapid Acting Agents
To help compensate for the delayed onset of action, different modifications were generated and evaluated in the peptide sequence of insulin. One modification that demonstrated improved efficacy involved switching Pro and Lys at positions B28 and B29 respectively. (Figure 4.7) This amino acid switch destabilized the hexadimerization interaction and resulted in rapid dissociation, which led to rapid absorption following injection. This resulting absorption profile is less than 15 min, and this version of insulin is called Insulin Lispro.
Another version that was generated is called Insulin Aspart where the Pro at position B28 was replaced with Asp. The newly introduced negative charge destabilizes the hexadimerizaiton interface, leading to a rapid absorption profile as seen with Insulin Lispro. Comparatively, Insulin Lispro has a slightly faster absorption rate and faster plasma peak time as well as rate of decline, although Insulin Aspart demonstrates slightly better stability. However, these differences are minor, and the real-world use of these products is relatively indistinguishable.
Insulin Glulisine is another version of insulin where Gly at B3 and Pro at B28 are replaced with Lys and Glu respectively. In addition to these modifications, the formulation contains polysorbate 20 instead of Zn2+. This becomes important because Zn2+ is required to maintain the insulin as hexamers. Without the hexamer formation, there is a significantly faster rate of absorption than Insulin Lispro or Insulin Aspart.
4.3.3 Intermediate Acting Agents
There are also relatively slower acting agents as well, with the most common version as Neutral Protamine Hagedorn (NHP) Insulin. In this case, the insulin molecule has the exact same sequence as naturally occurring insulin, but protamine is also included in the formulation. Protamine is a highly positively peptide and the introduction of this cation species creates a large, aggregated network of insulin hexamers. Once injected, insulin monomers need to be released from the hexameric structure, but also need to snake their way out of the mesh-like formulation. This leads a slow release of insulin over a period of hours, and NHP Insulin has a more delayed onset of action. NHP Insulin helps provides a steady low supply of insulin to the bloodstream, without repeated or continuous injections.
4.3.4 Long-Acting Agents
There are also agents that lead to significantly longer periods for onset of action, where the release of insulin is further delayed. These long-acting agents have similar pharmacokinetic profiles that lead to insulin release over 12-24 h. Insulin Glargine is the most prescribed long-acting agent (where A21 has Gly that replaces an Asn, and two Arg residues are added to the C-terminus of the B-chain). These changes lower the pI of the protein to 6.7, which results in precipitation at neutral pH as well as at the site of injection. Over time, Insulin Glargine will slowly re-enter solution and dissociate into monomers that can enter the blood stream.
There are other versions as well such as Insulin Determir (where a Thr at B30 is removed and a 14-C myristoyl fatty acid group is added) which creates additional binding to albumin proteins, and Insulin Degludec (where a Thr at B30 is removed and a 16-C fatty acid with Glu-spacer is added) which forms multi-hexamers and also binds albumin. In all cases, these versions add additional interactions that extend the timeframe required for insulin to dissociate into active monomers.
The different dissociation patterns of each type of insulin agent are shown in Figure 4.8.
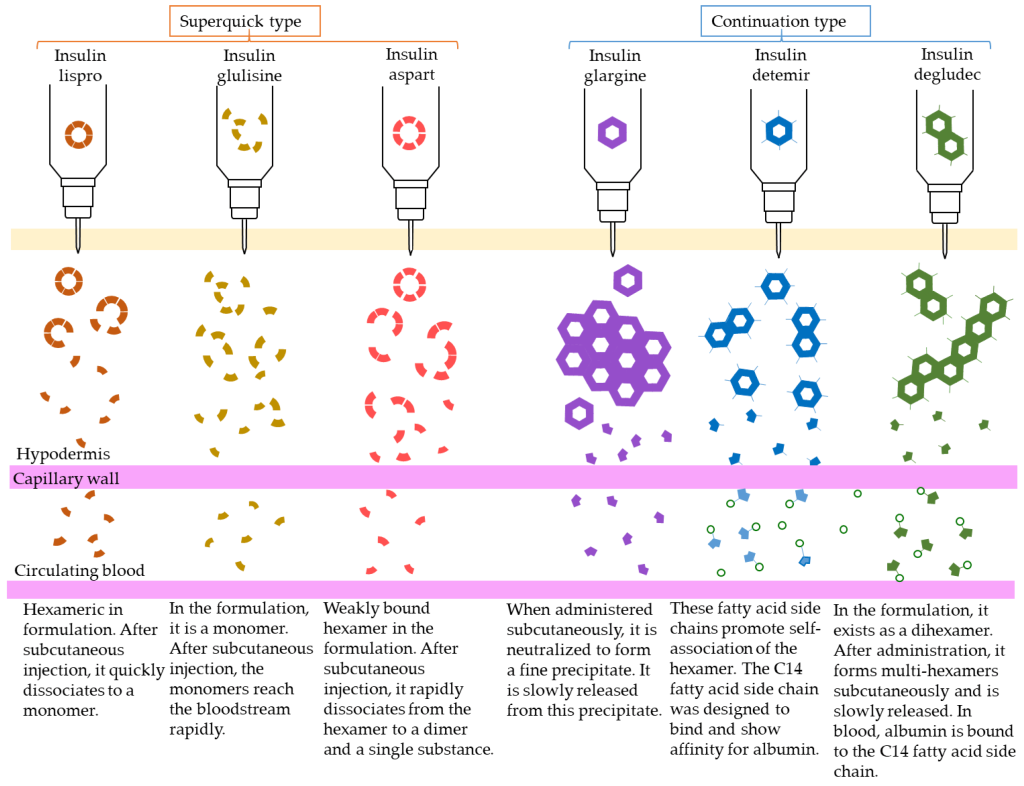
4.4 Treatments for Type II Diabetes
Importantly, the treatments for Type I diabetes all involve injections as they are large peptides that would not be suitable as oral agents since they would not survive in the stomach or be absorbed by small intestine without cleavage/modification. Type II diabetes usually emerges as a loss of sensitivity to insulin, and introduction of more insulin may not be the most efficient method to reduce blood sugar concentrations. In these cases, there have been a number of small molecules or oral agents developed.
4.4.1 Multiple Blood Glucose Lowering Effects: Biguanides
Biguanides were originally identified in the 1840s from herbs such as the French lilac, which is rich in guanidine derivatives. However, from an array of these guanidine compounds, several had significant adverse effects, and were not advanced. However, they were revisited again in the 1950s for treatment of diabetes, and the compound metformin was explored further. Chemically, a biguanide involves the linkage of two guanidine molecules, and metformin is capped with two methyl- groups on one end. Other compounds such as butformin (capped with a butyl group) or phenformin (capped with a phenyl group) presented with several side effects and were found to be less useful in treating diabetes. (Figure 4.9) Metformin is a relatively planar molecule, and is monoprotonated at neutral pH. It has approximately 50% oral bioavailability and a tmax of ~2.5 h. The mechanism of action for metformin is still heavily debated and it has been described to have at least three key effects:
- Reduced hepatic glucose formation (blocking gluconeogenesis)
- Reduced intestinal absorption of glucose
- Increased insulin sensitivity
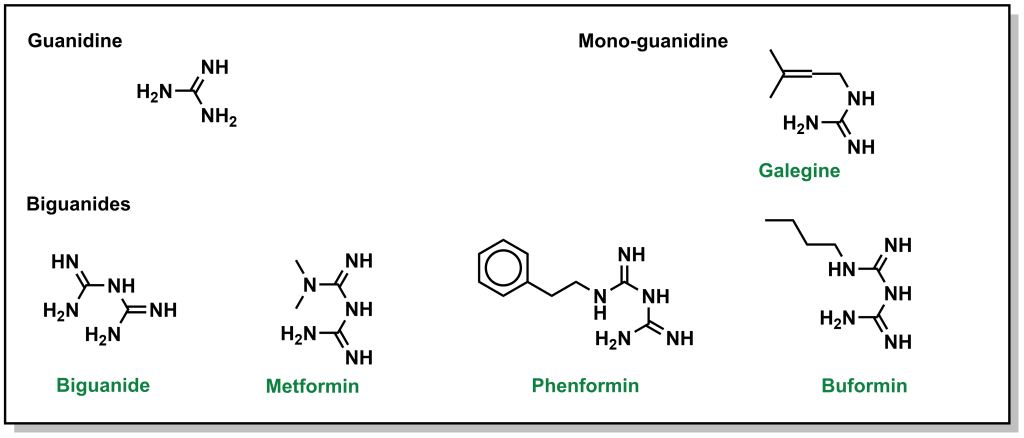
Physiological and biochemical studies have revealed that metformin enters the liver via action of OCT1 (organic cation transporter) and ultimately blocks the electron transport chain which is required for oxidative phosphorylation and energy generation. (Figure 4.10) Since the key molecule in electron shuttling and energy generation (NADPH) deposits electrons in the ETC to re-generate NADP+, shutting down the ETC leads to build up of NADPH in the cell. Excess NADPH causes multiple biochemical effects and signals the cells to stop converting glycogen to glucose. Additionally, the build up of NADPH can lead to NADPH reducing pyruvate to form lactic acid. As such, one of the potential adverse effects of metformin is lactic acidosis.
FIGURE 4.10 Different effects of metformin in the cell. Image Source: (Fig 1) by Ichiro Nojima and Jun Wada is used under a CC-BY 4.0 license.
Metformin is ultimately excreted by the kidneys and because of these effects, it is usually discontinued if a patient is admitted to the hospital for a condition where a CT scan may be likely. This is because the contrast agent is also processed by the kidneys. If both metformin and contrast agent are administered, since they are competing for renal clearance, metformin may remain in the body longer, and lead to excess concentrations and lactic acidosis.
4.4.2 Increasing Insulin Release: Sulfonylureas
Sulfonylureas are drugs that contain a sulfonylurea functional group (usually neighbouring an aryl ring) and bind the aptly named, sulfonylurea receptors (SURs) in the pancreas. (Fig 4.11) SUR proteins are ABC transporters that are part of a larger complex of ATP-sensitive potassium channels (called KATP channels) and act as gatekeepers of releasing insulin. These drugs have been utilized for several decades and are relatively inexpensive. Under normal function, the presence of glucose in the pancreas cells also leads to high concentration of intracellular ATP. High levels of ATP block the KATP channel, which leads to membrane depolarization triggering voltage gated Ca2+ channels and Ca2+ ion influx. As shown previously (Figure 4.3), the introduction of Ca2+ leads to insulin release.
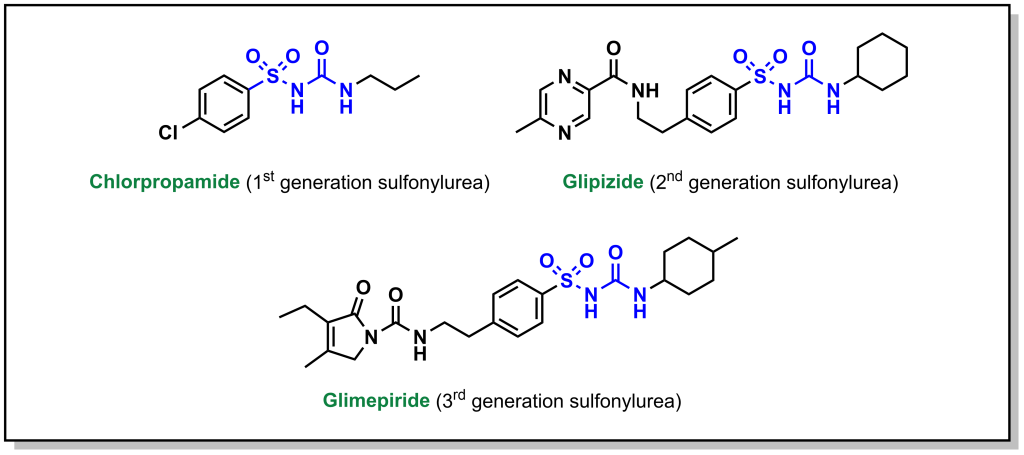
Administering sulfonylurea drugs produces the same response as high levels of intracellular ATP. Specifically, the sulfonylurea will bind the KATP channel, leading to membrane depolarization and Ca2+ influx. This essentially decouples the release of insulin from blood glucose concentrations. However, it should be noted that if excess sulfonylureas are administered, this can cause blood glucose concentrations to drop dramatically.
Some additional side effects of sulfonylureas are that they can cause the patient to gain weight (as sulfonylureas lead to additional insulin release, which triggers cells throughout the body to uptake more glucose). Furthermore, KATP channels are also located in other tissues in the body including the heart, and non-specific binding of sulfonylureas can lead to an increased risk of cardiac events.
4.4.3 Increasing Insulin Release: Meglitinides
Meglitinides have a similar mechanism of action as sulfonylureas – they bind to the KATP channel (at a different site on the SUR protein compared to sulfonylurea drugs). (Figure 4.12) However, the interaction between meglitinides and the SUR protein is not as strong as the sulfonylurea-SUR binding. This results in a relatively weaker effect that has a shorter duration of action. This reduced activity can be very useful, since it can lead to more flexibility in treatment options and meglitinides are not associated with adverse cardiovascular events. Meglitinides also present as a suitable option in case the patient is allergic to sulfonylureas.
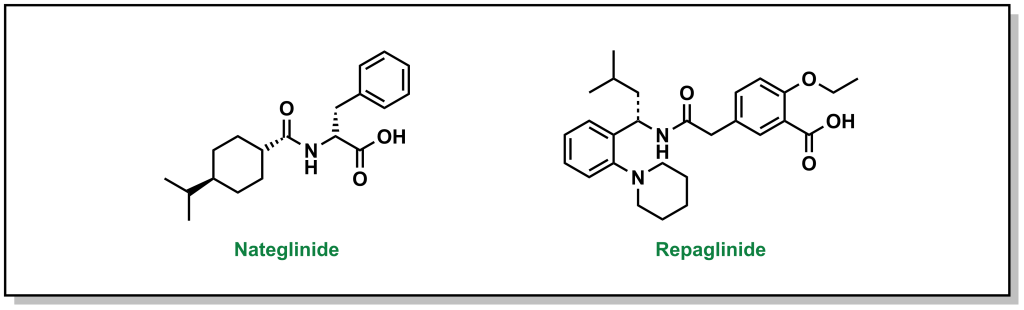
4.4.4 Increasing Insulin Production: Dipeptidyl Peptidase 4 (DPP-4) Inhibitors
In addition to the hormones produced by the pancreas, there are also hormones produced by the small intestine called incretins (including GIP and GLP1). These incretins stimulate the body to prepare for food intake as well as insulin production/release. These incretins are very rapidly degraded by dipeptidyl peptidase 4 (DDP-4). Prolonging the effects of GIP/GLP1 (by inhibiting DDP-4 with small molecules) can increase insulin levels in the body as well as other digestion and absorption effects. DDP-4 inhibitors engage with DPP-4 at the GLP1 interaction site, and they are considered protein-protein interaction inhibitors. Several drugs contain azolopyrimidines which are important for hydrogen bonding interactions within the binding site. (Figure 4.13)
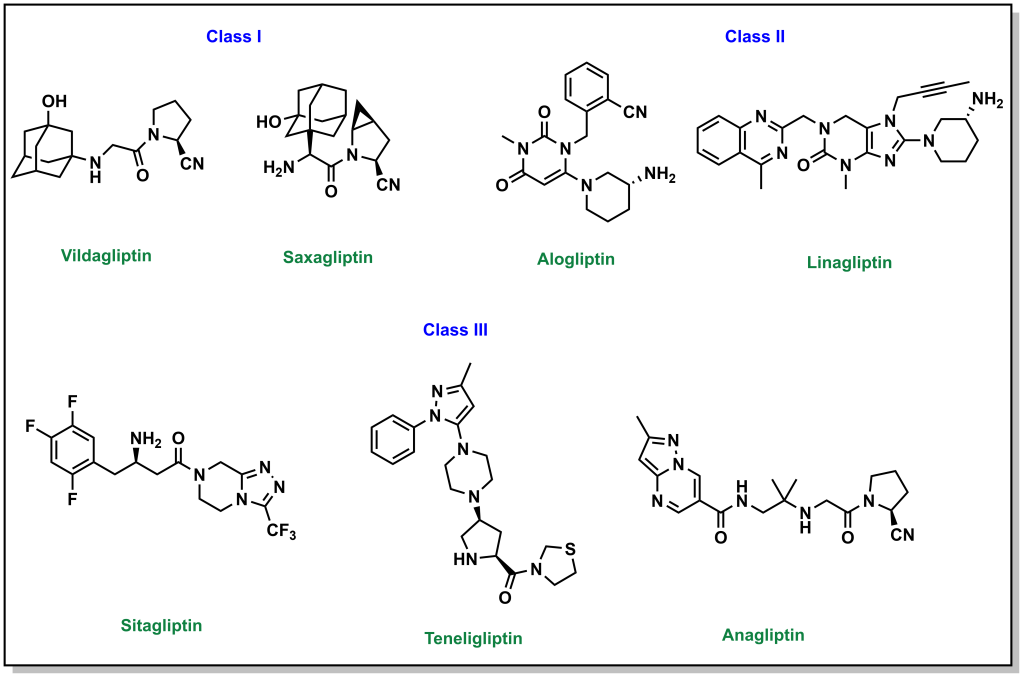
One side effect of these drugs is that they are usually associated with weight loss (although they lead to increased insulin release as with sulfonylureas ore meglitinides). This is because the prolonged incretin lifetime also increases feelings of satiety that usually suppresses diet and therefore reduce caloric intake.
4.4.5 Increasing Insulin Production: GLP1 Agonists
Similar to the mechanism with DDP-4 inhibitors, GLP1 agonists involve directly injecting the incretin GLP1 (comparable to injecting the hormone insulin in Type 1 Diabetes). GLP1 is preferred over GIP since it has more potent effects. There are two peptide backbones for these drugs, which are based on GLP1 and exendin-4 (originally isolated from the venom of the Gila monster reptile). (Figure 4.14) Since it is not a mammalian incretin, exendin-4 is not a substrate for DDP-4 and has longer life-time. As with DDP-4 inhibitors, these drugs slow the digestion of food, increase insulin output, and increase the feelings of satiety.

4.4.6 Blocking Glucose Absorption: α-Glucosidase Inhibitors
Absorption of carbohydrates occurs in the small intestine. However, blocking carbohydrate absorption can act as a preventative measure to reduce blood glucose concentrations. Large oligosaccharides cannot be directly absorbed and need to be cleaved into monosaccharides. This occurs by the action of α-glucosidases which are present at the small intestinal brush border. The drug, acarbose, is structurally very similar to oligosaccharides, but has a substantially higher affinity for α-glucosidase and is also non-cleavable. This leads to competitively blocking the active site of α-glucosidase, which prevents cleavage of oligosaccharides into monosaccharides, which subsequently blocks their absorption. These inhibitors can be more effective than traditional sulfonylureas, because they aim to prevent the spike in blood glucose levels proactively, as opposed to dealing with high concentrations after consumption.
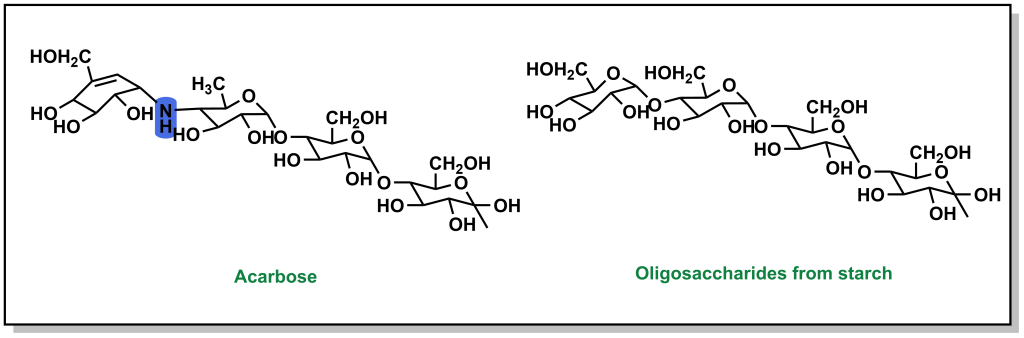
4.4.7 Blocking Glucose Re-absorption: SGLT-2 Inhibitors
Since Type 2 diabetic patients have a higher concentration of glucose in the blood, this will result in higher concentrations of glucose in the kidneys. The nephrons of the kidneys are designed to reabsorb glucose via sodium glucose L-transporters (largely performed by SGLT-2 transporters). (Figure 4.16) This function is physiologically beneficial since it helps retain nutrients. However, blocking these transporters in Type II diabetic patients can reduce blood glucose levels.
FIGURE 4.16 Reabsorption of glucose from the nephron of the kidney. Image Source: (Fig 1) by Daria M. Keller, Natasha Ahmed, Hamza Tariq, Malsha Walgamage, Thilini Walgamage, Azad Mohammed, Jadzia Tin-Tsen Chou, Marta Kałużna-Oleksy, Maciej Lesiak and Ewa Straburzyńska-Migaj is used under a CC-BY 4.0 license.
Phlorizin is the first drug for this purpose and consists of a glucose moiety linked to a system of two phenyl rings (the “aglycone” moiety) that is connected by an ethylene bridge. (Figure 4.17)
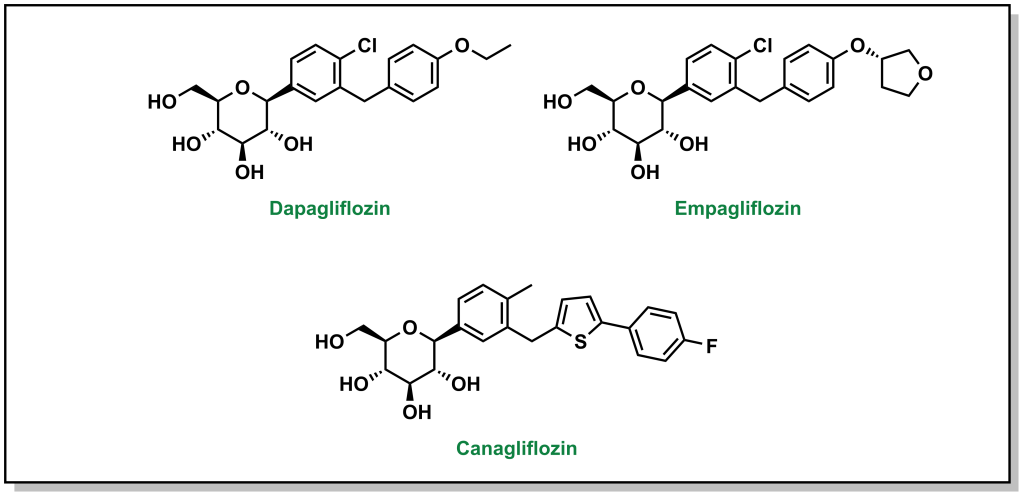
4.4.8 Indirect Blood Glucose Reduction: Blocking Triglycerides with Thiazolidinediones / Glitazones
Thiazolidinediones, also called glitazones, encourage cells to switch to carbohydrates / glucose as the principal energy source, which ultimately lowers blood glucose levels. (Figure 4.18)
FIGURE 4.18 Structures of thiazolidinediones.
These drugs are agonists for PPARγ (peroxisome proliferator-activated receptor gamma), which is a transcription factor that complexes with retinoid X receptor (RXR). The end result is increased gene expression that will store fatty acids (reducing free fatty acids in blood circulation) and causing an increased cellular dependence on glucose. GLUT4 is an additional gene target for PPARγ, leading to increased glucose into cells. (Figure 4.19) However, it should be noted that the increased fatty acid storage is also correlated with reduced osteoblast formation, and there is a decrease in bone mineral density and increase risk of fractures with prolonged use of thiazolidinediones.
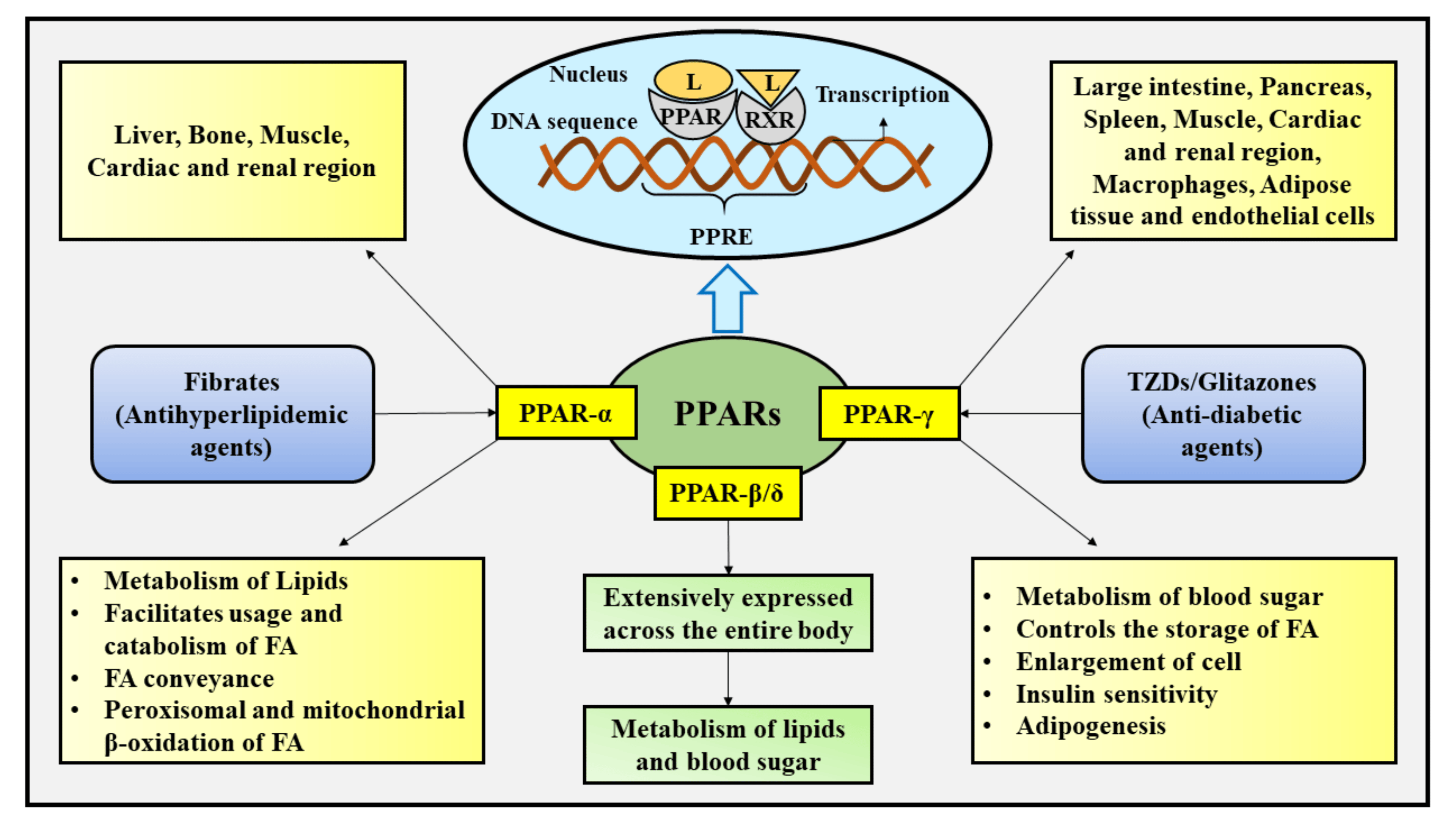
4.5 Summary
The following table provides a summary of treatments for Type II diabetes. The different types of insulins used for Type I diabetes are summarized in Figure 4.8.
Table 4.1 Pharmacological properties of Type II diabetes treatments.