3.3 – Oxidation of Alcohols
Introduction to Oxidations and Reductions
In organic chemistry, oxidation and reduction reactions can change the oxidation state of a carbon atom. An easy way to remember what oxidation and reduction reactions entail is that oxidation reactions result in either the gain of an electronegative atom (like oxygen, nitrogen or a halide) or loss of hydrogens atoms. In contrast, the term reduction is the opposite, and can be defined as the loss of an oxygen atom or gain of two hydrogen atoms.
Figure 3.3.a below shows how oxidation and reduction reactions can be used to convert a functional group between an alcohol, aldehyde, and carboxylic acid.
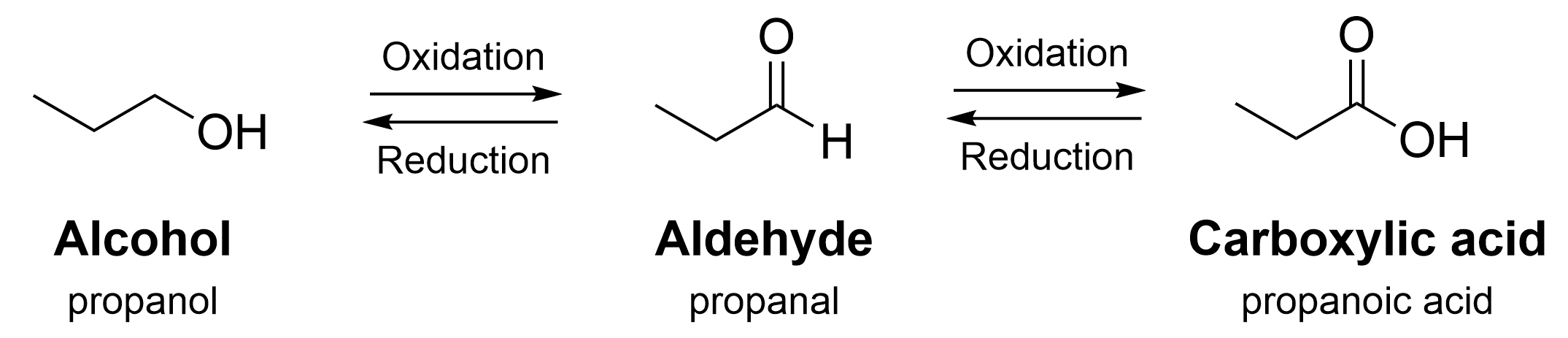
Oxidation of an alcohol or aldehyde increases the number of C–O bonds. while reduction of a carboxylic acid, an aldehyde, or ketone will decrease the number of C–O bonds. For example, in Figure 3.3.a, the alcohol (propanol) contains one C–O σ bond. The aldehyde (propanal) contains two C–O bonds, counting the σ bond and the π bond. The carboxylic acid (propanoic acid) contains three C–O bonds, including two σ bonds and one π bond.
Are You Wondering? The Differing Definitions of Oxidation and Reduction in Organic Chemistry & Electrochemistry
Oxidation states are a way of keeping track of electrons. When we calculate the oxidation state of an atom, we consider all bonds to be ionic, and distribute the electrons to the more electronegative atom. The oxidation numbers can then be calculated by subtracting the distributed number of electrons from the number of valence electrons on the atom.
For example, consider the combustion reaction below, with oxidation numbers assigned to each atom.
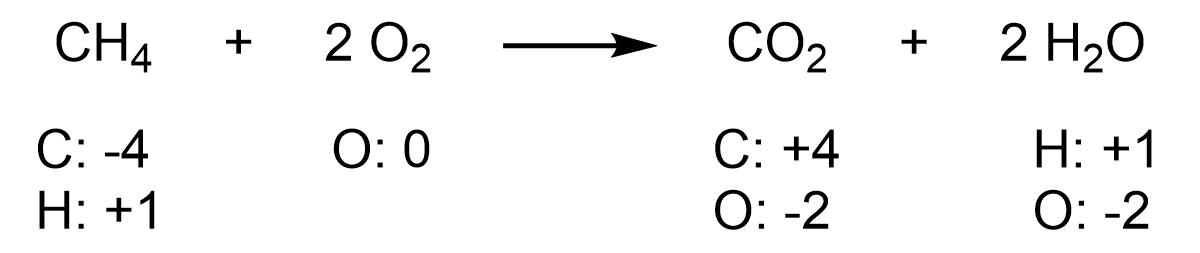
First, consider the oxidation numbers in methane, CH4. The electronegativities of C and H are 2.5 and 2.1, respectively, on the Pauling electronegativity scale. The oxidation numbers of C and H are calculated by assigning the electrons in each C–H bond to the more electronegative atom (carbon). This process renders each hydrogen atom with an oxidation number of +1, and the central carbon with an oxidation number of -4.
Next, consider the oxidation numbers in carbon dioxide, CO2. The electronegativities of C and O are 2.5 and 3.5, respectively, on the Pauling electronegativity scale. The oxidation numbers of C and O are calculated by assigning the electrons in each C–O bond to the more electronegative atom (oxygen). This process renders each oxygen atom with an oxidation number of -2, and the central carbon with an oxidation number of +4.
Thus, in this reaction, the oxidation number of carbon increases from -4 to +4, so carbon is being oxidized. At the same time, the oxidation number of oxygen decreases from 0 to -2, so oxygen is being reduced. Oxidation and reduction always occur together, whereby one element is oxidized, and another is reduced. However, an organic chemist would call this reaction an oxidation because they are focused on what is happening to the carbon-containing compound.
Oxygen is one of the most electronegative elements in the periodic table, and hydrogen is less electronegative than carbon. Therefore, a reaction that causes the number of C–O bonds to increase (or the number of C–H bonds to decrease) is considered an oxidation. Conversely, a reaction that causes the number of C–H bonds to increase (or the number of C–O bonds to decrease) is considered a reduction.
In conclusion, there are a few ways you can consider oxidation and reduction reactions:
- Electrochemistry method: Calculate the oxidation numbers of each element and determine which species is being oxidized and which is being reduced. This method may be tedious and complicated, especially for large molecules.
- Counting C–O bonds: The greater the number of C–O bonds (including both σ and π bonds), the more highly oxidized the compound. This method is faster and also works for large molecules.
Before discussing the oxidation of alcohols to carbonyls, recall how alcohols can be classified into one of three different categories: primary (1°), secondary (2°), and tertiary (3°) alcohols. To determine the classification of alcohol, focus on the carbon bonded to the hydroxyl group (Figure 3.3.b). If that carbon is bonded to one other carbon atom, then it is a primary alcohol – likewise, if bonded to two or three carbon atoms, it is a secondary or tertiary alcohol, respectively. This is important to understand as the degree of the alcohol will determine the reactivity and final products of the oxidation reaction.

Oxidizing Alcohols to Carbonyls
Figure 3.3.c displays the oxidation of a primary alcohol. The primary alcohol has one C–O σ bond and two C–H bonds that can be broken to oxidize the carbon. In the first oxidation reaction, the carbon atom of the alcohol becomes an aldehyde, which increases the number of C–O bonds to two, counting the σ bond and the π bond. As there is still another C–H bond that can be broken, a second oxidation reaction can occur. This results in the formation of a carboxylic acid, increasing the total number of C–O bonds to three, including two σ bonds and one π bond.
Comparing the number of C–O bonds, it can be said that alcohols are the least oxidized, as they only contain one C–O bond, whereas carboxylic acids are the most oxidized, containing three C–O bonds.
![Propanol, a primary alcohol, contains a carbon bonded to an ethyl group, an OH group and 2 other Hs, so it contains 1 C-O bond. A horizontal arrow to the right with [O] on top (oxidizing agent) forms an aldehyde (propanal) containing 2 C-O bonds. The alcohol carbon is double bonded to the oxygen and singly bonded to ethyl and H. One more oxidation leads to the conversion of aldehyde into a carboxylic acid (propanoic acid) with 3 C-O bonds. A large horizontal arrow drawn below the 3 compounds points towards the right where the alcohol on the extreme left is the least oxidized and the carboxylic acid is the most oxidized.](https://ecampusontario.pressbooks.pub/app/uploads/sites/3169/2023/06/Figure-3.3.c.-Oxidation-of-a-primary-alcohol-to-carboxylic-acid.png)
The same logic can be applied to the oxidation of secondary alcohols (Figure 3.3.d). The secondary alcohol has one C–O σ bond and one C–H bond that can be broken to oxidize the carbon. In the oxidation reaction, the carbon atom of the alcohol becomes a ketone, which increases the number of C–O bonds to two, counting the σ bond and the π bond. However, unlike aldehydes, ketones cannot undergo another oxidation reaction. The central carbon already contains two bonds to oxygen and two bonds to carbon. For a third C–O bond to form, a C–C bond must break. The mechanism of this reaction requires C–H σ bonds on the carbon bound to the oxygen atom for oxidations to occur. If no such C–H bonds exist, then the oxidation process cannot proceed
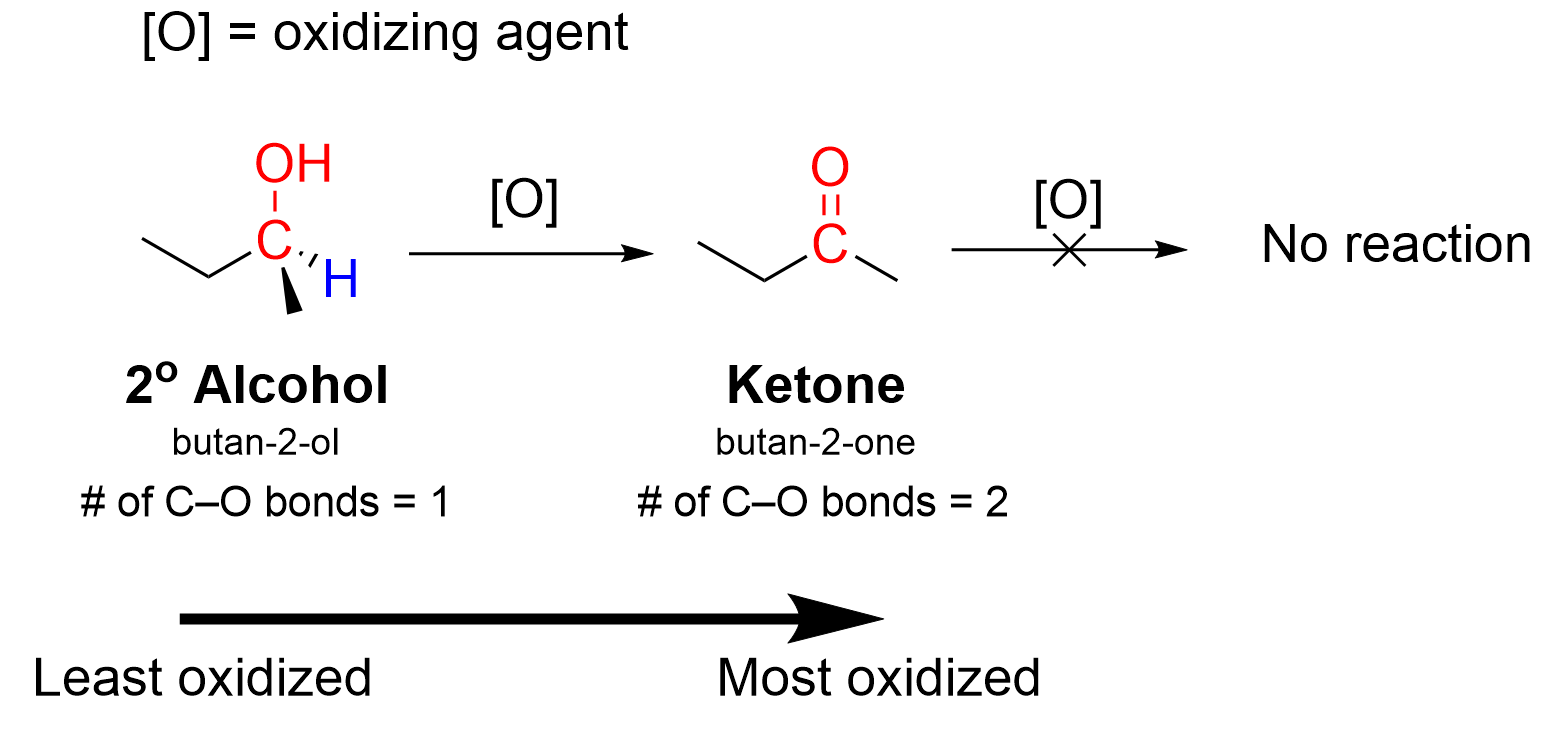
Tertiary alcohols are unable to undergo any form of oxidation (Figure 3.3.e). Tertiary alcohols contain three C–C bonds and no C–H bonds, meaning the oxidation would require breaking a C–C bond, which cannot occur.
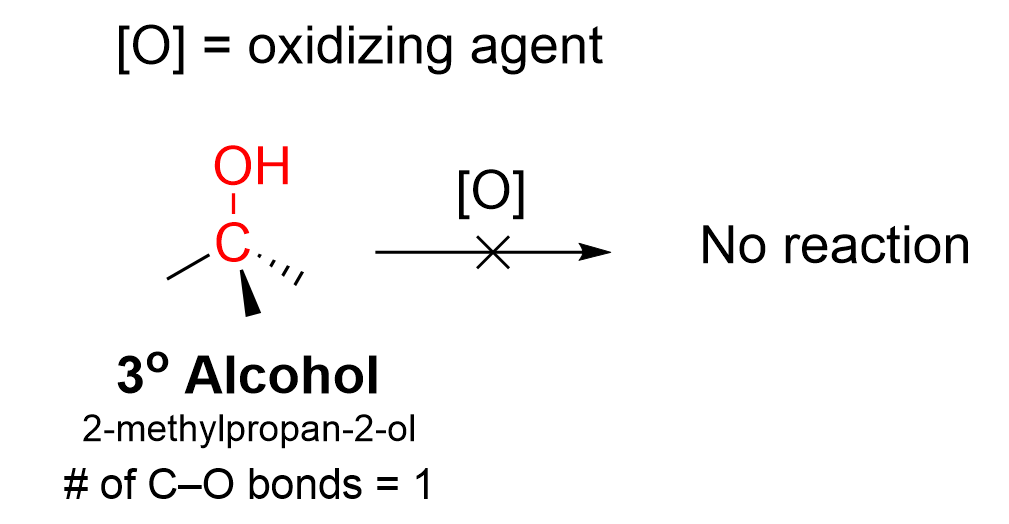
In summary, primary alcohols can undergo two oxidation events while secondary alcohols can undergo one oxidation event. Tertiary alcohols cannot be oxidized at all.
(The full solution to this problem can be found in Chapter 5.2)
Oxidizing Agents
Oxidizing agents are commonly metals with a high oxidation state. Examples of oxidizing agents include:
- potassium permanganate, KMnO4, which contains Mn(VII)
- potassium dichromate, K2Cr2O7 , which contains Cr(VI)
- pyridinium chlorochromate, denoted PCC, which contains Cr(VI)
Due to their high oxidation state, the metals (manganese or chromium) are easily reduced (gain electrons), but they must oxidize another species. In this case, the organic compound is oxidized as a result.
Oxidation reactions using KMnO4 or K2Cr2O7 are performed in water, in either acidic or basic conditions, to facilitate electron transfer. Using KMnO4 or K2Cr2O7 results in oxidizing a molecule to its highest possible oxidation state. For example, reacting KMnO4 with a primary alcohol produces a carboxylic acid. The aldehyde product cannot be isolated under most conditions. Similarly, reacting KMnO4 with a secondary alcohol yields a ketone.
Oxidation reactions using PCC are performed in an organic solvent (CH2Cl2) rather than water, so the reagent is often written as PCC in CH2Cl2. PCC is a more selective oxidizing agent in that it reacts with alcohols, but not with aldehydes. Thus, reacting PCC in CH2Cl2 with a primary alcohol will only perform the first oxidation to produce an aldehyde and will not oxidize further to a carboxylic acid. PCC can similarly oxidize secondary alcohols to produce ketones.
If a reaction calls for the conversion of an alcohol to an aldehyde, only PCC can be used. Reagents like KMnO4 or K2Cr2O7 cannot be used as they will oxidize the primary alcohol twice to yield a carboxylic acid. For a secondary alcohol, any of PCC, KMnO4, or K2Cr2O7 could be used to produce a ketone.
These oxidation reactions can be summarized below in Figure 3.3.f. You do not need to know the reaction mechanism of these oxidation reactions.
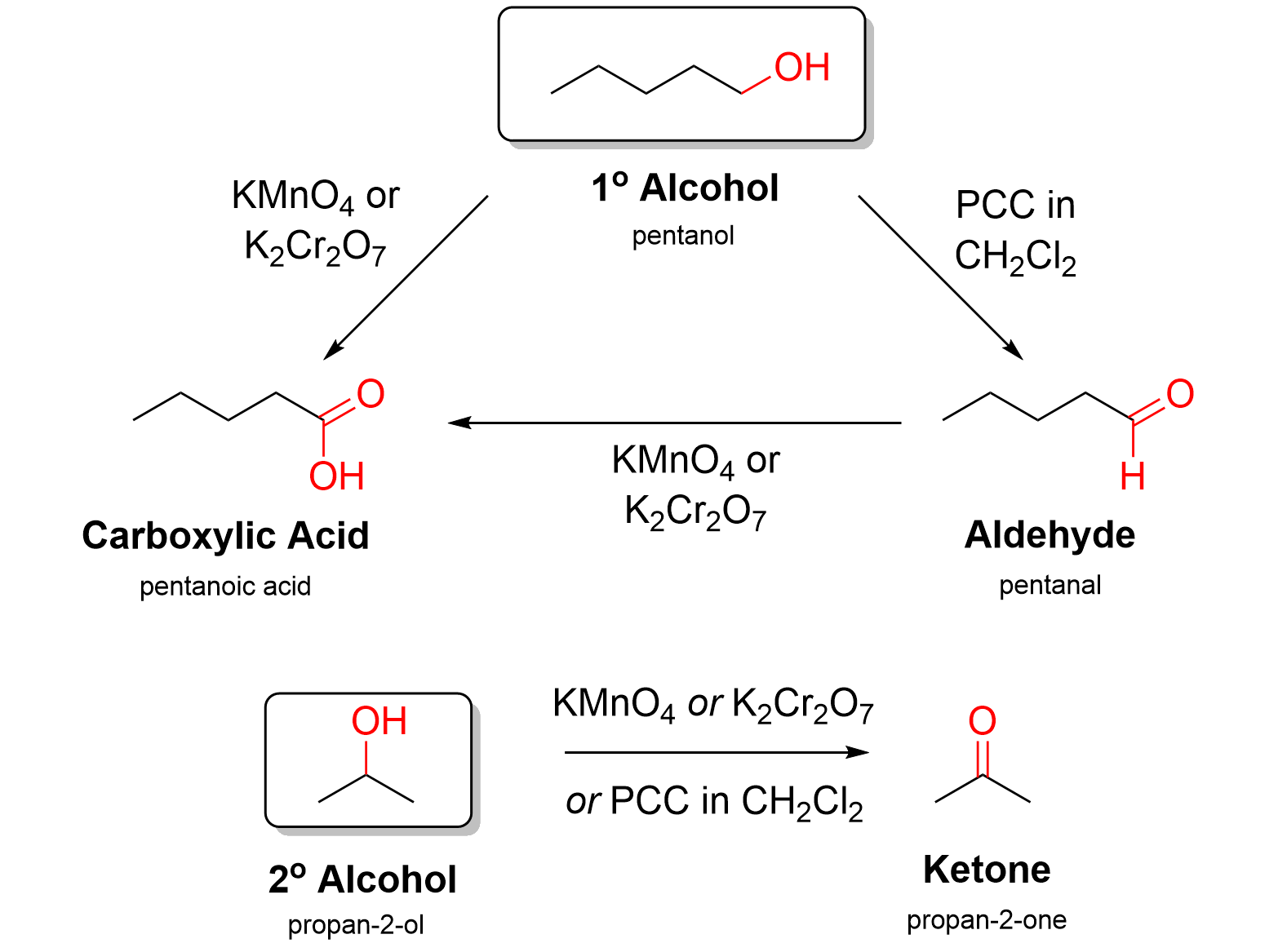
(The full solution to this problem can be found in Chapter 5.2)
Are You Wondering? More Sustainable Options for Oxidizing Compounds
Although we learn about oxidizing reagents through oxidizing agents such as PCC, chromium, and manganese, these methods are not very sustainable. Sustainable chemistry is a branch of chemistry that focuses on designing products and processes to minimize hazardous substances and byproducts, including the environmental impact of chemistry. Oxidation conditions including highly acidic environments and high heat levels tend to be unsafe, and reagents such as chromium are toxic carcinogens that can affect the user. Thus, it is imperative to develop more sustainable methods of oxidation that are safer and use less resources.
We can turn to biology to look for alternative methods of oxidation. Alcohol dehydrogenase is an enzyme found within our bodies that is oxidizes the alcohol we consume into acetic acid, a carboxylic acid. If the compound of interest is recognizable by the enzyme, alcohol dehydrogenase can be used for oxidation. This reaction takes place in water at near neutral pH as opposed to acidic conditions, making it a much safer and sustainable alternative as an enzyme catalyst is used rather than carcinogenic heavy metals.

However, this method is not perfect. Not all molecules can be recognized by the enzyme, as it must fit within the binding pocket for this to occur. Furthermore, not all molecules can be dissolved in water, which is the solvent for this reaction. Not all reactions are perfect, however, this is an alternative that can be used and is a step in the right direction to more sustainable chemistry practice.
Key Takeaways
- Primary and secondary alcohols can undergo oxidation reactions. Oxidation of an alcohol occurs when the carbon bound to the hydroxyl group gains a π bond to oxygen, and loses a σ bond to hydrogen.
- The reverse of an oxidation reaction is called a reduction reaction.
- Oxidation reactions of alcohols can yield an aldehyde, ketone or carboxylic acid, depending on the starting materials and oxidizing agent used.
- An oxidizing agent is required in oxidation reactions; it is a molecule which itself is highly oxidized.
- Three oxidizing agents are of interest: potassium permanganate (KMnO4), potassium dichromate (K2Cr2O7) and pyridinium chlorochromate (PCC) in CH2Cl2.
- KMnO4 and K2Cr2O7 are strong oxidizing agents. These will always produce the most oxidized product, i.e. they will fully oxidize a 1° alcohol to a carboxylic acid.
- PCC in CH2Cl2 is a selective oxidizing agent that will only oxidize a 1° alcohol to an aldehyde, or a 2° alcohol into a ketone.
Diversity in Chemistry: Mary Elliot Hill
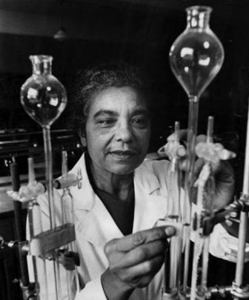
Although we only covered a handful of oxygen-containing functional groups, there are still numerous ones commonly employed in chemistry. For example, the ketone group is very similar to another functional group called a ketene, which consists of two subsequent C=C double bonds before the C=O bond (containing a structure of R-C=C=O). Mary Elliot Hill was a famous organic and analytical chemist who, alongside her husband, worked on the development of ketene synthesis which plays a significant role in plastic production. She also developed spectroscopic methods involving ultraviolet (UV) light to study ketene reaction progress. Hill graduated from Virginia State University with her bachelor’s degree in 1925, before becoming a teacher. She then went on to continue her education at the University of Pennsylvania and is believed to be one of the first African American women to be awarded a master’s degree in chemistry. Aside from her research, Hill was invested in education, instituting student chapters of the American Chemical Society at historically black colleges and universities where she taught. More information on Mary Elliot Hill can be found in this article celebrating her accomplishments.
Study Notes – Chapter 3.3
Any feedback or comments on this chapter? You may either email chemoer@mcmaster.ca, access this MS Form, or provide a comment in the feedback box below.