3.1.3 – SN1 Reaction Mechanisms
SN1 Reaction Mechanism
The previous section established how SN2 reactions cannot occur with tertiary alkyl halides as substrates due to the steric effect. However, they can undergo another type of nucleophilic substitution reaction called SN1.
SN1 reactions occur in more than one step by a dissociative mechanism where the leaving group leaves first. This generates a carbocation intermediate, which is a compound with a positively charged carbon atom that has trigonal planar geometry. The second step of an SN1 reaction involves nucleophilic attack by the electron rich nucleophile at the carbocation centre.
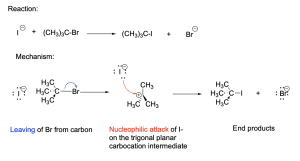
Let’s break down each step of the mechanism:

Step 1. The C–Br bond breaks with bromine acting as the leaving group. The transition state has tetrahedral geometry, and includes the C–Br bond in the process of breaking (depicted with a dashed line). This generates a positively charged carbocation intermediate, which has an empty p-orbital. The incomplete octet and positively charged carbon centre makes this intermediate highly reactive.
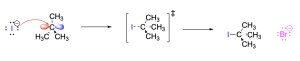
Step 2. The carbocation intermediate acts as an electrophile while the electron-rich iodide acts as the nucleophile. The iodide attacks the electron-deficient carbocation, donating its electron density into the empty p-orbital and forming a new C–I bond. The transition state has tetrahedral geometry and includes the C–I bond in the process of forming (depicted with a dashed line). This generates the final products: tert–butyl iodide and bromide.
Rate Law and Energy Diagram of SN1 Mechanism
The SN1 reaction proceeds through a two-step mechanism. This is shown in an energy profile diagram with two energy maxima, corresponding to the two transition state species. The local energy minimum represents the carbocation intermediate.
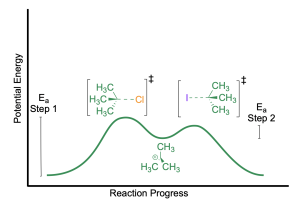
The first maximum represents the first step of the reaction: C–Br bond breaking and loss of the halide leaving group. This is the step with the largest activation energy barrier (Ea, step 1) in the mechanism. The activation energy barrier for the first step is largest because the initial reactant is stable as a neutral molecule with a complete octet. Bond breaking of the carbon-halide bond is endothermic, leading to a less stable, more reactive carbocation intermediate. The first step of an SN1 reaction is therefore the rate-determining step of the reaction.
The second maximum represents the second step of the reaction: nucleophilic attack by iodide to form the final product. This step has a lower activation energy (Ea, step 2) compared to the first step. The carbocation intermediate will readily accept electrons from the electron-rich nucleophile in an exothermic bond forming event. This yields the rate law shown below:
Rate = kobs[Alkyl Halide]
Since the first step is the slow step, the rate of an SN1 reaction only depends on the rate at which the carbon-halide breaks in the alkyl halide reactant. The reaction rate does not depend on the nucleophile. The overall rate law for an SN1 reaction is therefore first order, hence the name SN1 (Substitution Nucleophilic Unimolecular).
(The full solution to this problem can be found in Chapter 5.2)
The Effect of the Substrate Structure on the SN1 Reaction Rate
The alkyl halide substrate plays a key role in determining the rate of an SN1 reaction. It is experimentally observed that tertiary substrates most readily undergo an SN1 reaction, while methyl and primary alkyl halides are rarely observed to undergo an SN1 reaction.
The relative reactivity of substrates towards the SN1 reaction can be explained by the activation energy of the first step of the mechanism. This is in turn correlated to the energy of the carbocation intermediate: the more stable the carbocation intermediate, the lower the activation energy barrier to form that intermediate, and the faster it will form. In an SN1 reaction, tertiary substrates have the lowest activation energy barrier, and will undergo an SN1 reaction faster than secondary, primary and methyl substrates respectively.
An energy profile diagram of the four differently substituted alkyl halides (methyl, primary, secondary, and tertiary) is shown below:
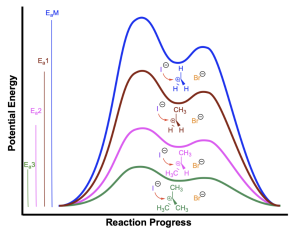
This trend is explained by the stability of the carbocation intermediates, which is in turn explained by considering the electron densities of surrounding groups. The greater the electron density surrounding the electron-deficient carbocation center, the greater the ability to dissipate the positive charge, and the more stable the carbocation. Alkyl groups possess larger electron clouds than hydrogen atoms and can donate their electron density towards the carbocation, and thereby dissipate the positive charge to a larger degree than hydrogen atoms.
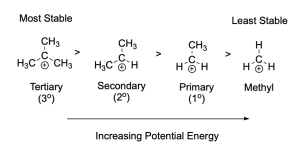
In the tertiary carbocation, the electron-deficient carbon center is surrounded by three alkyl groups, which donate some of their electron density towards the carbocation centre. This allows for dissipation of the positive charge, which stabilizes the carbocation. In the primary and secondary carbocations, there are fewer alkyl groups surrounding the carbocation center. As a result, these intermediates have less electron density surrounding the carbocation, which limits their stabilizing capacity. The methyl carbocation has only hydrogen atoms bound to the carbocation, resulting in limited electron density available to stabilize the carbocation center, making the methyl carbocation the least stable.
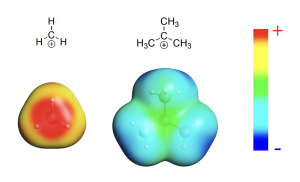
Nucleophilic Substitution – Making Alcohols and Ethers
An SN1 mechanism can also be used to synthesize alcohols and ethers, by reacting a tertiary alkyl halide substrate with either water or an alcohol, respectively, as the nucleophile. This reaction occurs in three steps, these being the two-steps involved in the SN1 mechanism, followed by a proton transfer step.
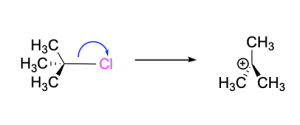
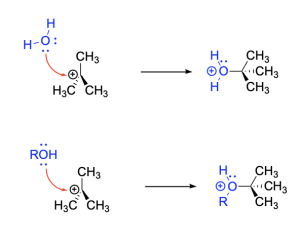
Step 2, top. Water acts as a nucleophile to attack the electrophilic carbocation center, generating an oxonium cation intermediate.
Step 2, bottom. An alcohol acts as a nucleophile to attack the electrophilic carbocation center, generating an oxonium cation intermediate.
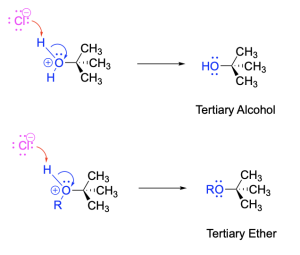
Step 3, top. Chloride (the halide leaving group from Step 1) acts as a base to deprotonate the oxonium intermediate resulting in HCl and a tertiary alcohol.
Step 3, bottom. Chloride (the halide leaving group from Step 1) acts as a base to deprotonate the oxonium intermediate, resulting in HCl and a tertiary ether.
The mechanism above in which the tertiary alkyl halide reacts with water or an alcohol, requires three steps. In comparison, the SN1 reaction examined earlier, in which the tertiary alkyl halide reacts with a halide such as iodide, requires two steps. This is because reaction with a neutral nucleophile (water or an alcohol) requires an extra deprotonation step (Step 3 in Figure 3.4.1.j.) to generate a final product; this step is not needed when using an anionic nucleophile (such as I–).
This reaction yields the energy profile diagram shown below:
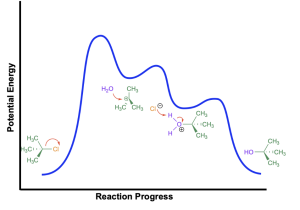
The first step of the reaction (loss of the halide leaving group) is the slow step and requires the greatest amount of energy. This will generate a carbocation intermediate. Then, water attacks the carbocation to form the corresponding oxonium intermediate. The second step has a lower activation energy than the first step because forming a full octet at carbon is favourable and will lead to stabilization. The third step involves deprotonation of the oxonium intermediate, yielding the neutral alcohol.
If an alcohol is used as the nucleophile (instead of water), then all steps are the same, except that the third step yields a neutral ether (instead of an alcohol).
The following video includes a worked example from a previous CHEM 1AA3 test or exam that students struggled with. Try solving it on your own before looking at the solution.
Key Takeaways
- SN1 stands for substitution nucleophilic unimolecular reaction
- This reaction is two steps, with the first step being the loss of a leaving group forming a carbocation intermediate, and the second step being the attack from a nucleophile
- The first step forms an unstable cationic intermediate, so it is the slow, rate limiting step with a large activation energy
- Tertiary alkyl halides form the most stable carbocation intermediate, as more alkyl groups will make it so more of their electron density is donated to the carbocation. This is why they are the most favoured for SN1 mechanisms, including making ethers and alcohols
Key terms in this chapter:
Key term | Definition |
Oxonium | A cation intermediate that is typically formed when water performs a nucleophilic attack on an electrophilic substrate. The oxygen atom will have a formal charge of +1. |
Any feedback or comments on this chapter? You may either email chemoer@mcmaster.ca, access this MS Form, or provide a comment in the feedback box below.
A cation intermediate that is typically formed when water performs a nucleophilic attack on an electrophilic substrate. The oxygen atom will have a formal charge of +1.