2.2 – Respiratory Structures and Their Function
The content of this chapter was adapted from the Concepts of Biology-1st Canadian Edition open textbook by Charles Molnar and Jane Gair (Chapter 20 -The respiratory system).
![]() |
2.2. Describe select respiratory structures in aquatic and terrestrial animals.2.3. Explain relationships between respiratory structure and function in generalized aquatic and terrestrial animal systems. |
Ventilation and Perfusion
Two important aspects of gas exchange in the lung are ventilation and perfusion. Ventilation is the movement of air into and out of the lungs, and perfusion is the flow of blood in the pulmonary capillaries. For gas exchange to be efficient, the volumes involved in ventilation and perfusion should be compatible. Therefore, ventilatory structures and perfusion surfaces have evolved to maximize this compatibility in both aquatic and terrestrial environment and examples of such structures and surfaces will be provided next.
Skin and gills
Fish and many other aquatic organisms have evolved gills to take up the dissolved oxygen from water (Figure 2.4). Gills are thin tissue filaments that are highly branched and folded. When water passes over the gills, the dissolved oxygen in water rapidly diffuses across the gills into the bloodstream. The circulatory system can then carry the oxygenated blood to the other parts of the body. In animals that contain coelomic fluid instead of blood, oxygen diffuses across the gill surfaces into the coelomic fluid. Gills are found in mollusks, annelids, and crustaceans.
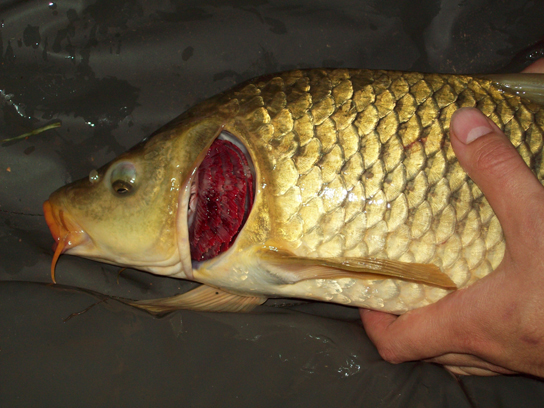
The folded surfaces of the gills provide a large surface area to ensure that the fish gets sufficient oxygen. Diffusion is a process in which material travels from regions of high concentration to low concentration until equilibrium is reached. In this case, blood with a low concentration of oxygen molecules circulates through the gills. The concentration of oxygen molecules in water is higher than the concentration of oxygen molecules in gills. As a result, oxygen molecules diffuse from water (high concentration) to blood (low concentration), as shown in Figure 2.5. Similarly, carbon dioxide molecules in the blood diffuse from the blood (high concentration) to water (low concentration).
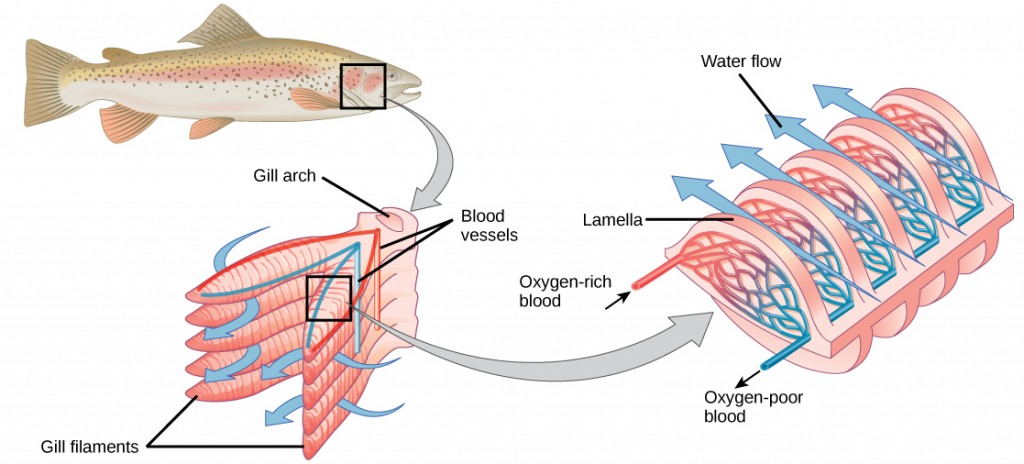
In terrestrial environments, animals showcase a diversity of respiratory system adaptations to obtaining oxygen (Figure 2.6), such as treachea in insects, alveoli in mammals and parabronchi in birds. You can also use the following gif animation as an overview of how air moves through respiratory system structured of a few terrestrial animals.
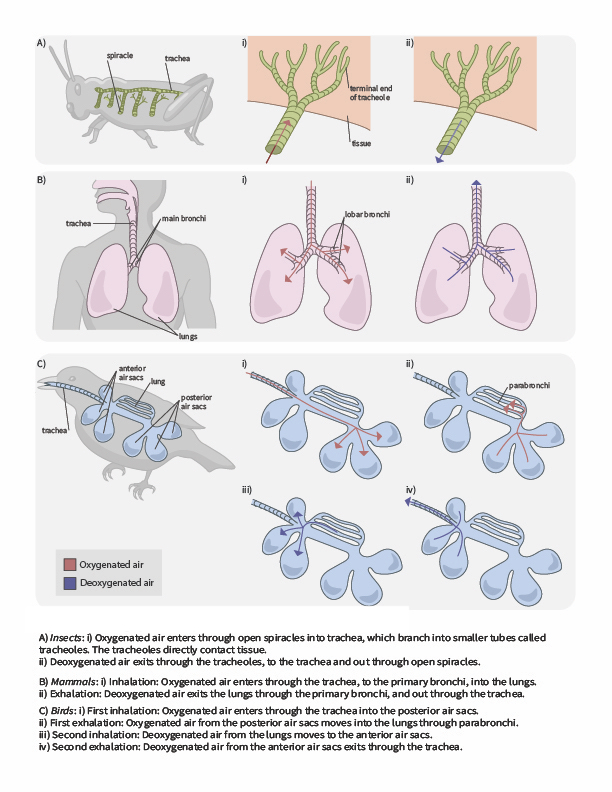
![]() |
Question 2.3
How would you describe gas exchange and breathing cycle in each of the examples you see in the gif animation? What are their similarities? What are their differences? |
Tracheal systems
Insect respiration is independent of its circulatory system; therefore, the blood does not play a direct role in oxygen transport. Insects have a highly specialized type of respiratory system called the tracheal system, which consists of a network of small tubes that carries oxygen to the entire body. The tracheal system is the most direct and efficient respiratory system in active animals. The tubes in the tracheal system are made of a polymeric material called chitin.
Insect bodies have openings, called spiracles along the thorax and abdomen. These openings connect to the tubular network, allowing oxygen to pass into the body (Figure 2.7) and regulating the diffusion of CO2 and water vapor. Air enters and leaves the tracheal system through the spiracles. Some insects can ventilate the tracheal system with body movements.
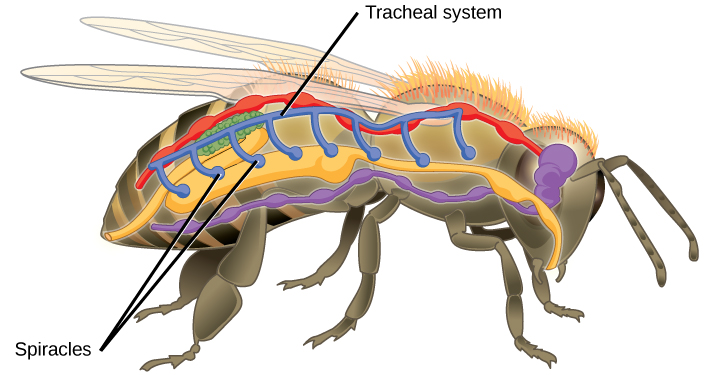
Mammalian systems
In mammals, pulmonary ventilation occurs via inhalation (breathing). During inhalation, air enters the body through the nasal cavity located just inside the nose (Figure 2.8). As air passes through the nasal cavity, the air is warmed to body temperature and humidified. The respiratory tract is coated with mucus to seal the tissues from direct contact with air. Mucus is high in water. As air crosses these surfaces of the mucous membranes, it picks up water. These processes help equilibrate the air to the body conditions, reducing any damage that cold, dry air can cause. Particulate matter that is floating in the air is removed in the nasal passages via mucus and cilia. The processes of warming, humidifying, and removing particles are important protective mechanisms that prevent damage to the trachea and lungs. Thus, inhalation serves several purposes in addition to bringing oxygen into the respiratory system.
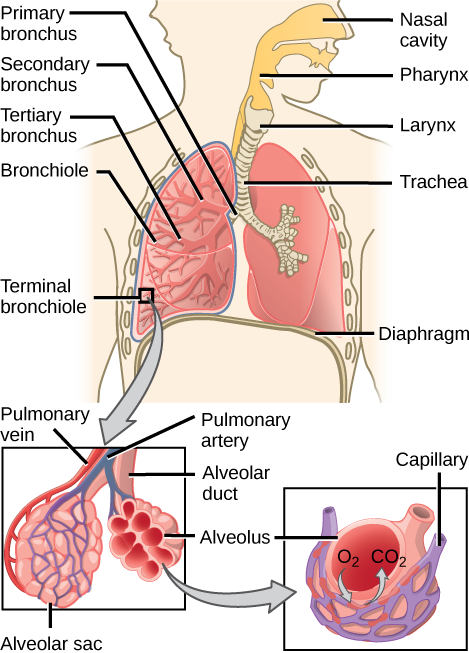
![]() |
Question 2.4
Which of the following statements about the mammalian respiratory system is false? |
From the nasal cavity, air passes through the pharynx (throat) and the larynx (voice box), as it makes its way to the trachea (Figure 2.9). The main function of the trachea is to funnel the inhaled air to the lungs and the exhaled air back out of the body. The human trachea is a cylinder about 10 to 12 cm long and 2 cm in diameter that sits in front of the esophagus and extends from the larynx into the chest cavity where it divides into the two primary bronchi at the mid-thorax. It is made of incomplete rings of hyaline cartilage and smooth muscle (Figure 2.9). The trachea is lined with mucus-producing goblet cells and ciliated epithelia. The cilia propel foreign particles trapped in the mucus toward the pharynx. The cartilage provides strength and support to the trachea to keep the passage open. The smooth muscle can contract, decreasing the trachea’s diameter, which causes expired air to rush upwards from the lungs at a great force. The forced exhalation helps to expel mucus when we cough. Smooth muscle can contract or relax, depending on stimuli from the external environment or the body’s nervous system.
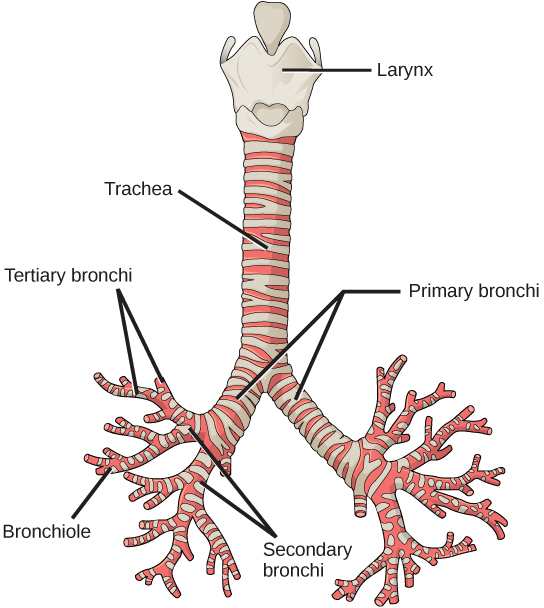
Lungs: bronchi and alveoli
The end of the trachea bifurcates (divides) to the right and left lungs. The lungs are not identical. The right lung is larger and contains three lobes, whereas the smaller left lung contains two lobes (Figure 2.10). The muscular diaphragm, which facilitates breathing, is inferior (below) to the lungs and marks the end of the thoracic cavity.
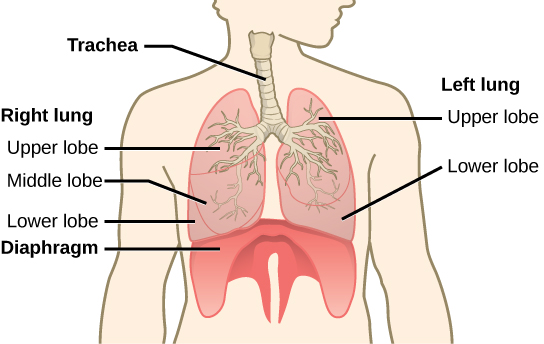
In the lungs, air is diverted into smaller and smaller passages or bronchi. Air enters the lungs through the two primary (main) bronchi (singular: bronchus). Each bronchus divides into secondary bronchi, then into tertiary bronchi, which in turn divide, creating smaller and smaller diameter bronchioles as they split and spread through the lung. Like the trachea, the bronchi are made of cartilage and smooth muscle. At the bronchioles, the cartilage is replaced with elastic fibers. Bronchi are innervated by nerves of both the parasympathetic and sympathetic nervous systems that control muscle contraction (parasympathetic) or relaxation (sympathetic) in the bronchi and bronchioles, depending on the nervous system’s cues. In humans, bronchioles with a diameter smaller than 0.5 mm are the respiratory bronchioles. They lack cartilage and therefore rely on inhaled air to support their shape. As the passageways decrease in diameter, the relative amount of smooth muscle increases.
The terminal bronchioles subdivide into microscopic branches called respiratory bronchioles. The respiratory bronchioles subdivide into several alveolar ducts. Numerous alveoli and alveolar sacs surround the alveolar ducts. The alveolar sacs resemble bunches of grapes tethered to the end of the bronchioles (Figure 2.11). In the acinar region, the alveolar ducts are attached to the end of each bronchiole. At the end of each duct are approximately 100 alveolar sacs, each containing 20 to 30 alveoli that are 200 to 300 microns in diameter. Gas exchange occurs only in alveoli. Alveoli are made of thin-walled parenchymal cells, typically one-cell thick, that look like tiny bubbles within the sacs. Alveoli are in direct contact with capillaries (one-cell thick) of the circulatory system. Such intimate contact ensures that oxygen will diffuse from alveoli into the blood and be distributed to the cells of the body. In addition, the carbon dioxide that was produced by cells as a waste product will diffuse from the blood into alveoli to be exhaled. The anatomical arrangement of capillaries and alveoli emphasizes the structural and functional relationship of the respiratory and circulatory systems. Because there are so many alveoli (~300 million per lung) within each alveolar sac and so many sacs at the end of each alveolar duct, the lungs have a sponge-like consistency. This organization produces a very large surface area that is available for gas exchange. The surface area of alveoli in the lungs is approximately 75 m2. This large surface area, combined with the thin-walled nature of the alveolar parenchymal cells, allows gases to easily diffuse across the cells.
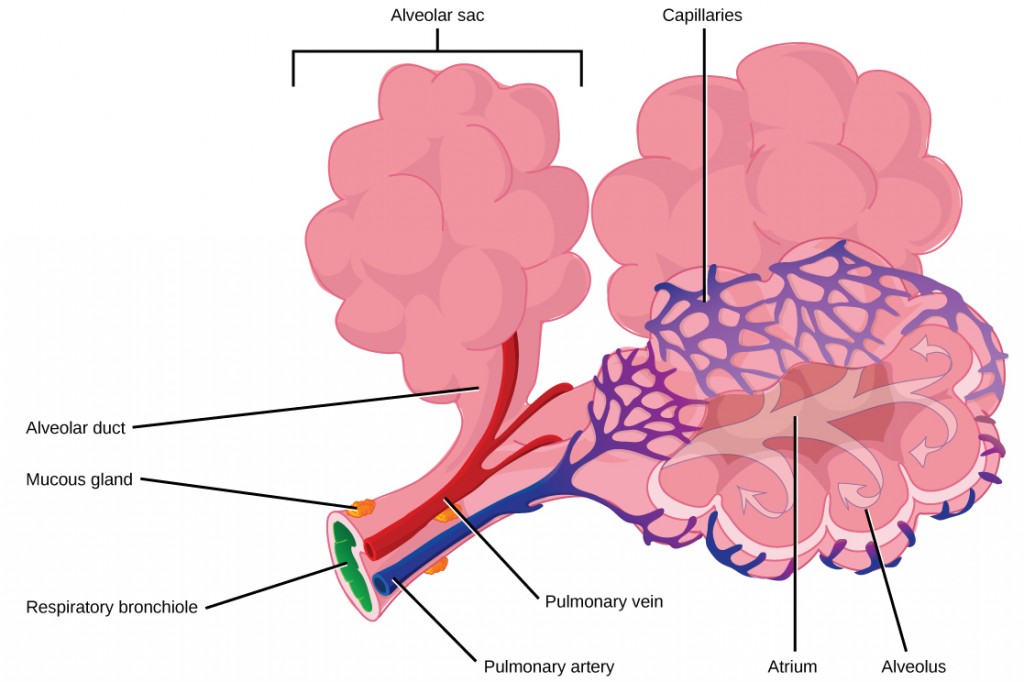
![]() |
Question 2.5
Which of the following statements about the human respiratory system is false? |
![]() |
For further interest and comparison of human lungs with other animal respiratory systems, here is a link to an interesting article. |
Pulmonary ventilation is the act of breathing, which can be described as the movement of air into and out of the lungs. The major mechanisms that drive pulmonary ventilation are atmospheric pressure (Patm); the air pressure within the alveoli, called intra-alveolar pressure (Palv); and the pressure within the pleural cavity, called intrapleural pressure (Pip).
Mechanisms of breathing
The intra-alveolar and intrapleural pressures are dependent on certain physical features of the lung. However, the ability to breathe—to have air enter the lungs during inspiration and air leave the lungs during expiration—is dependent on the air pressure of the atmosphere and the air pressure within the lungs.
Pressure relationships
Inspiration (or inhalation) and expiration (or exhalation) are dependent on the differences in pressure between the atmosphere and the lungs. In a gas, the pressure is a force created by the movement of gas molecules that are confined. For example, a certain number of gas molecules in a two-liter container has more room than the same number of gas molecules in a one-liter container (Figure 2.13). In this case, the force exerted by the movement of the gas molecules against the walls of the two-liter container is lower than the force exerted by the gas molecules in the one-liter container. Therefore, the pressure is lower in the two-liter container and higher in the one-liter container. At a constant temperature, changing the volume occupied by the gas changes the pressure, as does changing the number of gas molecules. Boyle’s law describes the relationship between volume and pressure in a gas at a constant temperature. Boyle discovered that the pressure of a gas is inversely proportional to its volume: If volume increases, pressure decreases. Likewise, if volume decreases, pressure increases. Pressure and volume are inversely related (P = k/V). Therefore, the pressure in the one-liter container (one-half the volume of the two-liter container) would be twice the pressure in the two-liter container. Boyle’s law is expressed by the following formula:
In this formula, P1 represents the initial pressure and V1 represents the initial volume, whereas the final pressure and volume are represented by P2 and V2, respectively. If the two- and one-liter containers were connected by a tube and the volume of one of the containers were changed, then the gases would move from higher pressure (lower volume) to lower pressure (higher volume).

Pulmonary ventilation is dependent on three types of pressure: atmospheric, intra-alveolar, and intrapleural. Atmospheric pressure is the amount of force that is exerted by gases in the air surrounding any given surface, such as the body. Atmospheric pressure can be expressed in terms of the unit atmosphere, abbreviated atm, or in millimeters of mercury (mm Hg). One atm is equal to 760 mm Hg, which is the atmospheric pressure at sea level. Typically, for respiration, other pressure values are discussed in relation to atmospheric pressure. Therefore, negative pressure is pressure lower than the atmospheric pressure, whereas positive pressure is the pressure that it is greater than the atmospheric pressure. A pressure that is equal to the atmospheric pressure is expressed as zero.
Intra-alveolar pressure (intrapulmonary pressure) is the pressure of the air within the alveoli, which changes during the different phases of breathing (Figure 2.14). Because the alveoli are connected to the atmosphere via the tubing of the airways (similar to the two- and one-liter containers in the example above), the intrapulmonary pressure of the alveoli always equalizes with the atmospheric pressure.

Intrapleural pressure is the pressure of the air within the pleural cavity, between the visceral and parietal pleurae. Similar to intra-alveolar pressure, intrapleural pressure also changes during the different phases of breathing. However, due to certain characteristics of the lungs, the intrapleural pressure is always lower than, or negative to, the intra-alveolar pressure (and therefore also to atmospheric pressure). Although it fluctuates during inspiration and expiration, intrapleural pressure remains approximately –4 mm Hg throughout the breathing cycle.
Competing forces within the thorax cause the formation of the negative intrapleural pressure. One of these forces relates to the elasticity of the lungs themselves—elastic tissue pulls the lungs inward, away from the thoracic wall. Surface tension of the alveolar fluid, which is mostly water, also creates an inward pull of the lung tissue. This inward tension from the lungs is countered by opposing forces from the pleural fluid and thoracic wall. Surface tension within the pleural cavity pulls the lungs outward. Too much or too little pleural fluid would hinder the creation of the negative intrapleural pressure; therefore, the level must be closely monitored by the mesothelial cells and drained by the lymphatic system. Since the parietal pleura is attached to the thoracic wall, the natural elasticity of the chest wall opposes the inward pull of the lungs. Ultimately, the outward pull is slightly greater than the inward pull, creating the –4 mm Hg intrapleural pressure relative to the intra-alveolar pressure. Transpulmonary pressure is the difference between the intrapleural and intra-alveolar pressures, and it determines the size of the lungs. A higher transpulmonary pressure corresponds to a larger lung.
Physical factors affecting ventilation
In addition to the differences in pressures, breathing is also dependent upon the contraction and relaxation of muscle fibers of both the diaphragm and thorax. The lungs themselves are passive during breathing, meaning they are not involved in creating the movement that helps inspiration and expiration. This is because of the adhesive nature of the pleural fluid, which allows the lungs to be pulled outward when the thoracic wall moves during inspiration. The recoil of the thoracic wall during expiration causes compression of the lungs. Contraction and relaxation of the diaphragm and intercostals muscles (found between the ribs) cause most of the pressure changes that result in inspiration and expiration. These muscle movements and subsequent pressure changes cause air to either rush in or be forced out of the lungs.
Other characteristics of the lungs influence the effort that must be expended to ventilate. Resistance is a force that slows motion, in this case, the flow of gases. The size of the airway is the primary factor affecting resistance. A small tubular diameter forces air through a smaller space, causing more collisions of air molecules with the walls of the airways. The following formula helps to describe the relationship between airway resistance and pressure changes:
As noted earlier, there is surface tension within the alveoli caused by water present in the lining of the alveoli. This surface tension tends to inhibit expansion of the alveoli. However, pulmonary surfactant secreted by type II alveolar cells mixes with that water and helps reduce this surface tension. Without pulmonary surfactant, the alveoli would collapse during expiration.
Thoracic wall compliance is the ability of the thoracic wall to stretch while under pressure. This can also affect the effort expended in the process of breathing. In order for inspiration to occur, the thoracic cavity must expand. The expansion of the thoracic cavity directly influences the capacity of the lungs to expand. If the tissues of the thoracic wall are not very compliant, it will be difficult to expand the thorax to increase the size of the lungs.
Gas laws and air composition
Gas molecules exert a force on the surfaces with which they are in contact; this force is called pressure. In natural systems, gases are normally present as a mixture of different types of molecules. For example, the atmosphere consists of oxygen, nitrogen, carbon dioxide, and other gaseous molecules, and this gaseous mixture exerts a certain pressure referred to as atmospheric pressure (Table 2.1). Partial pressure (Px) is the pressure of a single type of gas in a mixture of gases. For example, in the atmosphere, oxygen exerts a partial pressure, and nitrogen exerts another partial pressure, independent of the partial pressure of oxygen (Figure 2.15). Total pressure is the sum of all the partial pressures of a gaseous mixture. Dalton’s law describes the behavior of nonreactive gases in a gaseous mixture and states that a specific gas type in a mixture exerts its own pressure; thus, the total pressure exerted by a mixture of gases is the sum of the partial pressures of the gases in the mixture.
Table 1. Partial and total pressures of a gas.
Partial Pressures of Atmospheric Gases | ||
---|---|---|
Gas | Percent of total composition | Partial pressure
(mm Hg) |
Nitrogen (N2) | 78.6 | 597.4 |
Oxygen (O2) | 20.9 | 158.8 |
Water (H2O) | 0.4 | 3.0 |
Carbon dioxide (CO2) | 0.04 | 0.3 |
Others | 0.06 | 0.5 |
Total composition/total atmospheric pressure | 100% | 760.0 |

Partial pressure is extremely important in predicting the movement of gases. Recall that gases tend to equalize their pressure in two regions that are connected. A gas will move from an area where its partial pressure is higher to an area where its partial pressure is lower. In addition, the greater the partial pressure difference between the two areas, the more rapid is the movement of gases.
Solubility of gases in liquids
Henry’s law describes the behavior of gases when they come into contact with a liquid, such as blood. Henry’s law states that the concentration of gas in a liquid is directly proportional to the solubility and partial pressure of that gas. The greater the partial pressure of the gas, the greater the number of gas molecules that will dissolve in the liquid. The concentration of the gas in a liquid is also dependent on the solubility of the gas in the liquid. For example, although nitrogen is present in the atmosphere, very little nitrogen dissolves into the blood because the solubility of nitrogen in blood is very low. The exception to this occurs in scuba divers; the composition of the compressed air that divers breathe causes nitrogen to have a higher partial pressure than normal, causing it to dissolve in the blood in greater amounts than normal. Too much nitrogen in the bloodstream results in a serious condition that can be fatal if not corrected. Gas molecules establish an equilibrium between those molecules dissolved in liquid and those in the air.
The composition of air in the atmosphere and in the alveoli differs. In both cases, the relative concentration of gases is nitrogen > oxygen > water vapor > carbon dioxide. The amount of water vapor present in the alveolar air is greater than that in atmospheric air (Table 2.2). Recall that the respiratory system works to humidify incoming air, thereby causing the air present in the alveoli to have a greater amount of water vapor than atmospheric air. In addition, alveolar air contains a greater amount of carbon dioxide and less oxygen than atmospheric air. This is no surprise, as gas exchange removes oxygen from and adds carbon dioxide to the alveolar air. Both deep and forced breathing cause the alveolar air composition to be changed more rapidly than during quiet breathing. As a result, the partial pressures of oxygen and carbon dioxide change, affecting the diffusion process that moves these materials across the membrane. This will cause oxygen to enter and carbon dioxide to leave the blood more quickly.
Table 2.2. Partial and total pressures of alveolar gases.
Composition and Partial Pressures of Alveolar Air | ||
---|---|---|
Gas | Percent of total composition | Partial pressure
(mm Hg) |
Nitrogen (N2) | 74.9 | 569 |
Oxygen (O2) | 13.7 | 104 |
Water (H2O) | 6.2 | 40 |
Carbon dioxide (CO2) | 5.2 | 47 |
Total composition/total alveolar pressure | 100% | 760.0 |
Overall, the partial pressure of oxygen in alveolar air is about 104 mm Hg, whereas the partial pressure of the oxygenated pulmonary venous blood is about 100 mm Hg. When ventilation is sufficient, oxygen enters the alveoli at a high rate, and the partial pressure of oxygen in the alveoli remains high. In contrast, when ventilation is insufficient, the partial pressure of oxygen in the alveoli drops. Without the large difference in partial pressure between the alveoli and the blood, oxygen does not diffuse efficiently across the respiratory membrane. The body has mechanisms that counteract this problem. In cases when ventilation is not sufficient for an alveolus, the body redirects blood flow to alveoli that are receiving sufficient ventilation. This is achieved by constricting the pulmonary arterioles that serves the dysfunctional alveolus, which redirects blood to other alveoli that have sufficient ventilation. At the same time, the pulmonary arterioles that serve alveoli receiving sufficient ventilation vasodilate, which brings in greater blood flow. Factors such as carbon dioxide, oxygen, and pH levels can all serve as stimuli for adjusting blood flow in the capillary networks associated with the alveoli.
Ventilation is regulated by the diameter of the airways, whereas perfusion is regulated by the diameter of the blood vessels. The diameter of the bronchioles is sensitive to the partial pressure of carbon dioxide in the alveoli. A greater partial pressure of carbon dioxide in the alveoli causes the bronchioles to increase their diameter as will a decreased level of oxygen in the blood supply, allowing carbon dioxide to be exhaled from the body at a greater rate. As mentioned above, a greater partial pressure of oxygen in the alveoli causes the pulmonary arterioles to dilate, increasing blood flow.
![]() |
Question 2.6
Gas moves from an area of ________ partial pressure to an area of ________ partial pressure. |
![]() |
Question 2.7
The partial pressure of carbon dioxide is 45 mm Hg in the blood and 40 mm Hg in the alveoli. What happens to the carbon dioxide? |
![]() |
Question 2.8 A smoker develops damage to several alveoli that then can no longer function. How does this affect gas exchange? |