Chapter 3: G protein-Coupled Receptors (GPCRs) in signalling pathways
3.1 Introduction
GPCRs are a group of receptors that play key physiological roles in biological processes, responding to cues such as aromatic compounds, neurotransmitters, hormones, and even light. These stimuli are relayed as a response to the interior of the cell. In contrast to RTKs, GPCRs activate G proteins (guanine-nucleotide binding proteins) which also forms the basis for their name. This module will focus on structural and functional aspects of GPCRs.
3.2 Chapter Specific Learning Outcomes
Upon successful completion of this chapter, you will be able to:
- Describe the structure of GPCRs
- Explain how the structural properties dictate isolation of GPCRs
- Distinguish between two classes of G proteins
- Describe the subunit composition of heterotrimeric G proteins
- Demonstrate how the Gα subunit functions analogous to a molecular switch
- Outline the general steps in the GPCR signalling pathway
- Differentiate between first and second messenger, providing an example for each
- Illustrate downstream effects in the GPCR pathway
- List the reaction catalyzed by Adenylyl Cyclase (AC)
- Describe how the enzyme AC can regulate gene expression
- Interpret signalling pathways
3.3 Structural and Functional Properties of GPCRs
3.3.1 Describe the canonical structure of GPCRs.
GPCRs are integral proteins (similar to enzyme coupled receptors or RTKs), that are embedded within the plasma membrane. The classical GPCR structure consists of seven transmembrane α-helices (numbered H1-H7) (Figure 3.1). The helical portions are connected by extracellular or intracellular loops. Ligands can engage with a GPCR by binding to the extracellular portion (located near at the N-terminus). The C-terminus (carboxyl portion) is situated in the cytosol and also contains an additional α-helix (H8). Importantly, GPCRs also associate with a G-protein binding domain in the cytosolic region.
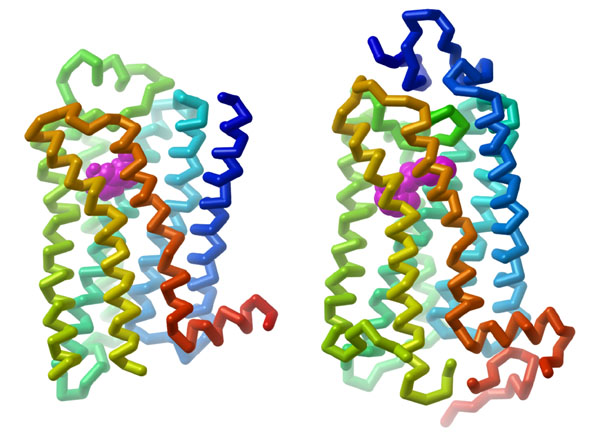
3.3.2 Based on their structural properties, which of the following reagents would be most effective to isolate GPCRs from the plasma membrane?
a. Treatments with lipids and detergents (e.g. sodium dodecyl sulfate – SDS).
b. Incubation with low salt concentration solution (e.g. sodium chloride).
c. Increasing the pH of the buffer.
Since GPCRs contain several transmembrane helices, they require a hydrophobic environment in order to preserve their structural integrity. Due to the nature of the proteins (tightly associated with the membrane), treatment with detergents or lipid bicelles can assist with isolation of GPCRs (Weis & Kobilka, 2018). The detergents containing hydrophobic regions can interact with both the membrane and the transmembrane helices. This helps remove the GPCR from the membrane, while still maintaining the overall structure by capturing it within a lipid containing particle.
3.3.3 Which of the following techniques is preferred to determine the structure of the adrenergic receptor?
a. X-ray crystallography
b. Fluorescence microscopy
c. Differential scanning calorimetry
X-ray crystallography is a common biophysical technique to reveal protein structure information with atomic-level resolution. This technique uses X-rays that are directed in a focused beam towards a protein ‘crystal’. The X-rays will be diffracted as they pass through the crystal and produce a specific diffraction pattern (or shadow). This diffraction pattern can be mathematically deconvoluted to solve for the position of the different atoms in the crystal, which provides the three-dimensional structure of the protein.
A protein will ‘crystalize’ when water is removed from the protein sample in a very specific manner. Experimentally, this can be extremely challenging but it is a powerful technique that provides high resolution images of the atoms of the protein. Each protein will produce an unique crystal that is dependent on its three-dimensional structure.
3.3.4 List common ligands which activate GPCRs.
There are a diverse number of ligands that can activate GPCRs including specific peptides, nucleotides, and photons. However, GPCRs also possess endogenous activity, albeit at a lower level compared to the presence of a ligand. Ligands which promote GPCR activity above the basal state are referred to as agonists (Weis & Kobilka, 2018). Some ligands can bind to a GPCR and block the response, which are referred to as antagonists.
There are more than 900 GPCRs identified in the human proteome and each one has a different ligand (Gaitonde & González-Maeso, 2017). Moreover, GPCRs represent targets for ~30% of all drugs that are available on the market (Hauser et al., 2017). Specific examples of endogenous GPCR ligands include epinephrine, norepinephrine, histamine, glucagon, calcitonin, oxytocin, and neurokinin.
3.3.5 Where is the ligand binding site on GPCRs?
Ligands such as peptides bind in close proximity to the amino terminus or extracellular loops. Conversely, small ligands bind within the transmembrane heptahelical region (Calebiro et al., 2021).
3.4 GPCR mediated signalling pathways
3.4.1 Describe features of a G protein and how it is related to a GPCR.
G proteins are guanine nucleotide-binding proteins that function as intermediary signalling proteins between GPCRs and secondary messengers. These proteins can bind both GTP and GDP which determines whether they are active (GTP-bound) or inactive (GDP-bound).
G proteins can be partitioned into two categories: i) small monomeric G proteins and ii) large heterotrimeric G proteins. Large heterotrimeric G proteins are composed of three different protein subunits: α (Gα), β (Gβ) and γ (Gγ). Both the α and γ subunits are connected to the membrane through lipid anchors (which are long hydrophobic tails that interact with the membrane). All three subunits exist in a trimeric complex including a bound GDP molecule at the Gα subunit. Upon activation of the GPCR (ligand binding), the GDP is exchanged for a GTP. This leads to dissociation of the Gα subunit from the Gβ and Gγ subunits. Both the α and βγ subunits can associate with additional proteins to facilitate signal transduction.
GPCRs can also associate with small G proteins. These proteins are more similar to the Gα subunits of heterotrimeric G proteins and also have GTP and GDP binding capabilities. The small G proteins are most commonly recognized as Ras GTPases.
3.4.2 Predict a likely consequence for GPCR if a non-hydrolyzable analogue (such as GMP·PNP) was present in high concentrations inside the cell compared to GTP.
a. Gα would be able to bind GMP·PNP however hydrolysis would not occur. Therefore, Gα would remain in the ‘on’ or active state.
b. Gα would be able to bind GMP·PNP and eventually convert to GMP. Therefore Gα would be converted in the ‘off’ or inactive state.
The α subunit of the heterotrimeric G protein possesses a guanine nucleotide binding site. Therefore, the α subunit can bind to GMP-PNP, especially considering the concentration is higher relative to GTP (Maegley et al., 1996) (Figure 3.2).
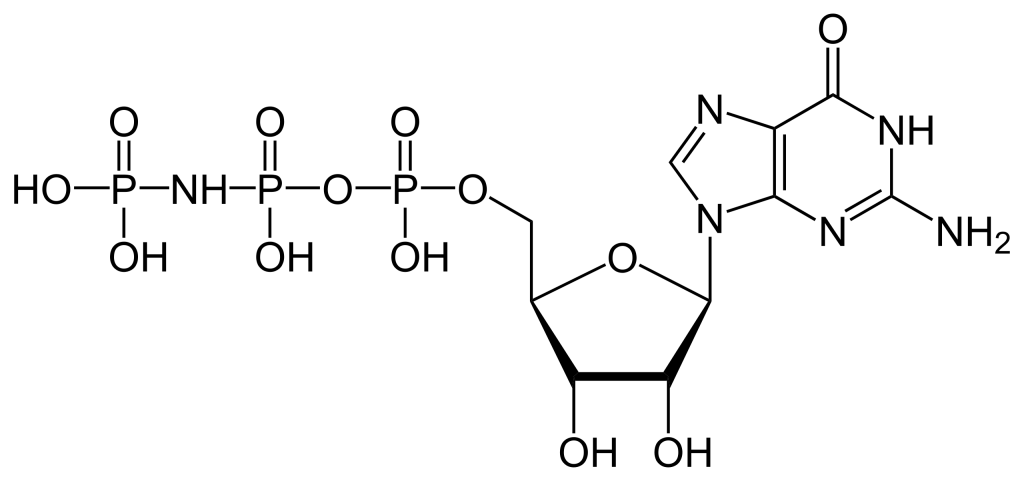
Conventionally, when the αβγ complex is bound to GDP, this results in the inactive state. The βγ subunits together stabilize the α subunit in the inactive state. When the αβγ complex is bound to GTP, this results in the active state, eventually leading to Gα dissociation.
3.4.3 Which G protein subunit functions as a molecular switch?
a. Gα
b. Gβ
c. Gγ
The Gα subunit functions as a molecular switch possessing a GTPase domain.
3.4.4 Which component would be referred to as a GEF?
a. GPCR
b. Gα
c. Gβ
d. Gγ
A GEF represents a guanine nucleotide exchange factor which enables exchanging GDP for GTP to promote GPCR activation. During the GPCR signalling pathway, a ligand will bind to the receptor on the extracellular surface. This triggers a conformational change that is propagated through the transmembrane helices and results in the intracellular side of the GPCR having GEF activity. Therefore, the activated GPCR helps to exchange GDP for GTP at the Gα subunit resulting in Gα activation.
3.4.5 Describe the signalling mechanism for representative GPCR.
During a period of stress, the body can react by releasing hormones such as adrenaline (or also referred to as epinephrine) which helps to surmount an appropriate response to external cues such as the fight or flight response.
The hormone signal (adrenaline) binds to the adrenergic receptor, causing a conformational change (Figure 3.3). The receptor associates and binds with the G protein located in the cytoplasmic space. The Gα subunit (shown in orange) is associated with GDP and binding to the activated GPCR triggers GEF activity and the GDP is replaced with GTP. The GTP binding leads to dissociation of the Gα and Gβγ (shown in yellow and green). The dissociated Gα (bound to GTP) interacts with an effector, such as a kinase to stimulate a downstream effect. In this pathway, the effector is the enzyme, adenylyl cyclase (AC), a transmembrane protein shown in blue.
Adenyl cyclase catalyzes the conversion of ATP to cAMP which is a powerful signalling molecule, called a secondary messenger (Figure 3.3). As the levels of the second messenger in the cytoplasm increase, the cAMP interacts with a number of proteins including protein kinase A (PKA). The nucleotide, cAMP activates PKA and PKA will subsequently phosphorylate a number of target proteins leading to activation of multiple proteins involved in glucose and lipid metabolism, thereby providing additional energy for the body.
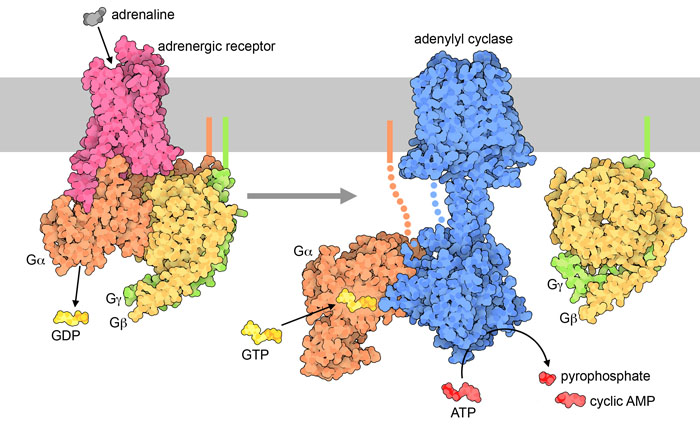
3.4.6 Which reaction does the enzyme adenylyl cyclase catalyze?
The enzyme adenylyl cyclase or adenylate cyclase is analogous to guanylyl cyclase. It catalyzes the conversion of ATP to the cyclized version (cyclic AMP – cAMP). A molecule of pyrophosphate is also released (Figure 3.3).
3.4.7 Differentiate between a primary messenger and secondary messenger.
The extracellular signal is an example of a primary messenger, while secondary messengers are typically part of downstream signalling events. For example, the ligand that binds to a GPCR (such as epinephrine) is classified as a primary messenger. The primary messenger generally does not cross the cell membrane. Once the primary messenger binds to a target (such as a GPCR), the signal is transmitted to another molecule. In the case of epinephrine and the adrenergic receptor, cAMP is produced, and it is an example of a secondary messenger. Besides small, diffusible molecules, ions are another common example of secondary messengers. The process of converting one signal to another is referred to as signal transduction, and this facilitates responses to different stimuli. Importantly, the primary messenger can trigger the production or release of multiple secondary messenger molecules, which substantially amplifies the signal.
3.4.8 Why is PKA also referred to as cAMP-dependent protein kinase?
The regulatory mechanism for activation of PKA involves cAMP molecules. Structurally, PKA is a heterotetramer composed of two catalytic subunits and two regulatory subunits (Figure 3.4). The catalytic subunits harbour the active site for protein phosphorylation (and will bind ATP as well as the substrate) as well as a regulatory subunit binding domain. The regulatory subunits possess an auto-inhibitory domain (that acts as a substrate analog to bind and inhibit the kinase activity of the catalytic subunit), as well as a cAMP binding domain and a dimerization domain. Regulatory subunits exist in different isoforms, and the dimerization can be covalent via disulfide linkages or non-covalent.
In the absence (or low concentrations) of cAMP, the regulatory subunit dimer is associated with the catalytic PKA subunits, which maintains the proteins in an inactive conformation. In the presence of cAMP (or increasing concentrations), the cAMP will bind to the regulatory subunits at the cAMP binding domain, leading to allosteric modulation and dissociation of the catalytic subunits. The free PKA catalytic subunits are now capable of binding and phosphorylation downstream targets in the cell.
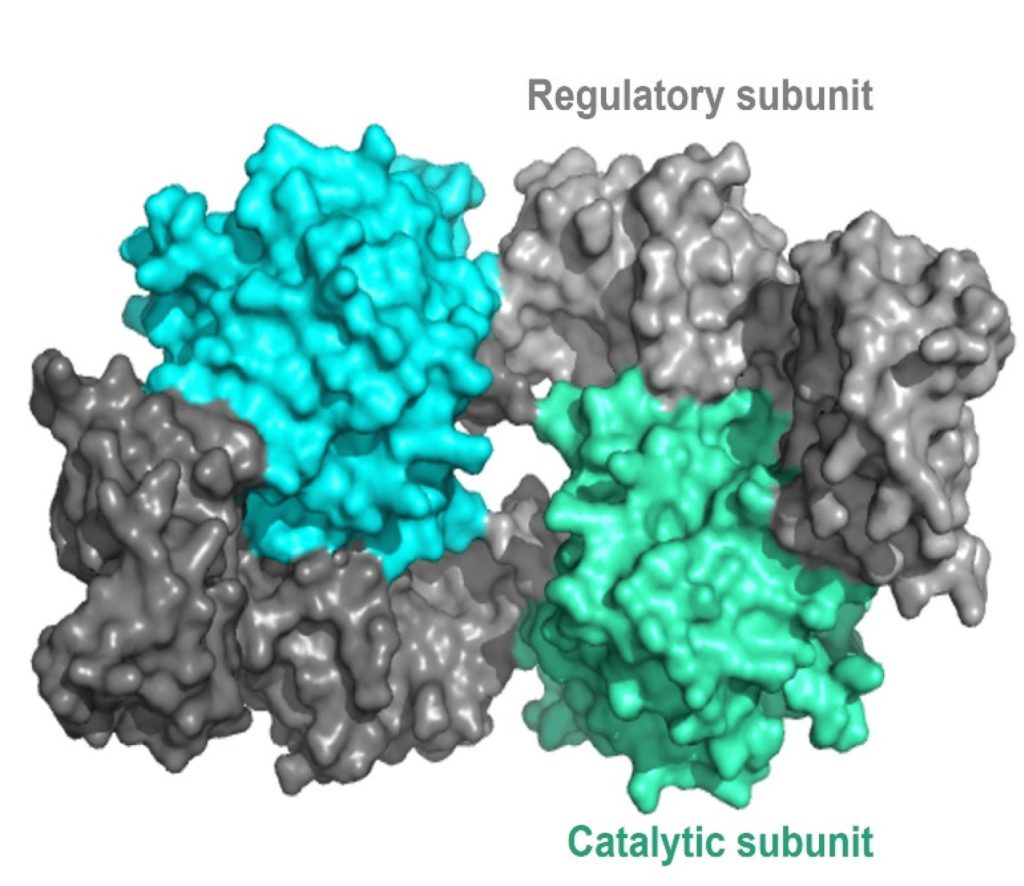
3.4.9 Which amino acids are phosphorylated by PKA on corresponding target proteins?
a. Ser
b. Thr
c. Tyr
d. a and b
PKA is a Ser/Thr kinase. However, PKA is often localized to the cell membrane through its association with a scaffold protein, A-kinase anchoring protein (AKAP) (Figure 3.5) (Welsh et al., 2023). In some cases, this introduces a layer of spatial targeting for the kinase and another mechanism for regulation.
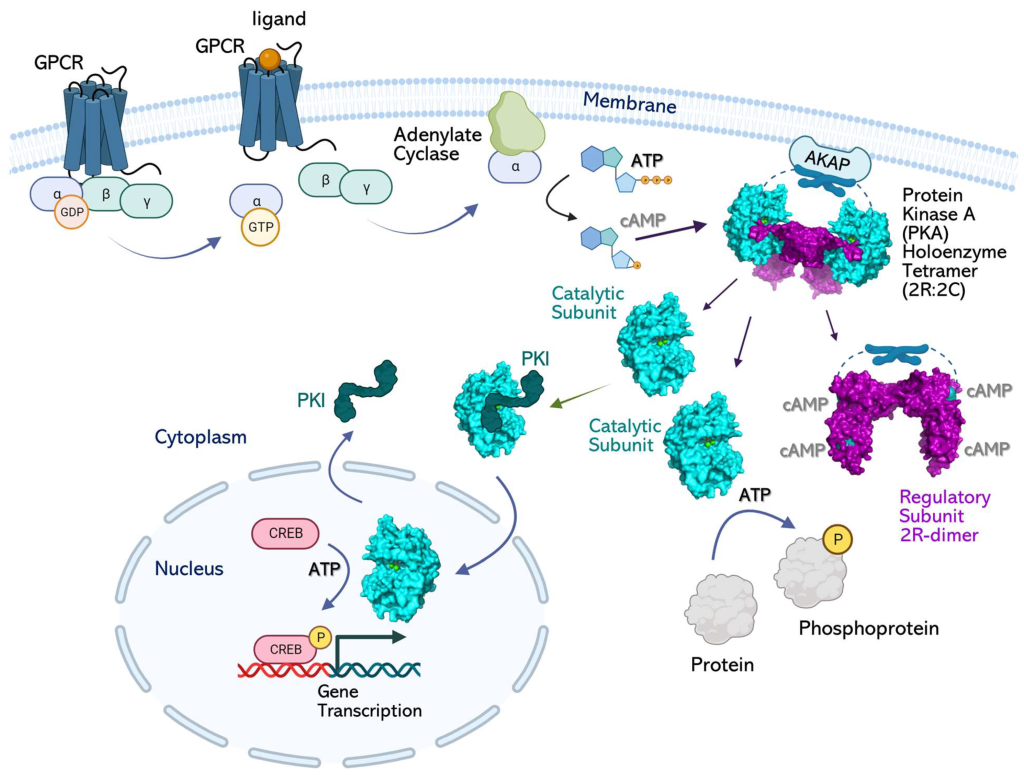
3.4.10 How is AC linked to regulation of gene expression?
Adenyl cyclase leads to increased production of cAMP, which (as described above) activates PKA. The catalytic subunits of PKA may no longer be anchored to the membrane (if not associated with lipid anchoring proteins) and can diffuse to other regions of the cell. This includes the ability to enter the nucleus and regulate gene expression. Within the nucleus PKA phosphorylates a Ser on different transcription factors such as CRE-binding protein (CREB) (Figure 3.5). CREB can bind to another protein CBP (CREB binding protein) and the CREB/CBP complex binds to CRE (cAMP Response Element), a sequence of DNA which flanks genes under its control, including neuropeptides (e.g. somatostatin, enkephalin), tyrosine hydroxylase, and other proteins involved in circadian rhythms (Montminy et al., 1990).
3.4.11 What is an effector protein?
This refers to proteins downstream of the pathway altered by the signal. For example, a GPCR is usually not an effector protein, but adenylyl cyclase is an effector protein. Adenylyl cyclase performs the downstream activity and carries out the “effect” of the original signal (ligand-binding event).
3.4.12 How can the α subunit influence the activity of the downstream effector, adenylyl cyclase?
There are a variety of α subunits responsible for different physiological responses. For example, the αs (also referred to as Gαs) protein stimulates the enzyme adenylyl cyclase. Conversely, the inhibitor G protein α subunit – αi or Gαi protein binds and inhibits the enzyme adenylyl cyclase.
3.4.13 How are GPCRs linked to a different cellular response, such as opening Ca2+ channels?
Similar to the β-adrenergic receptor (where epinephrine activates the GPCR), different ligands (such as the hormone angiotensin II) bind and activate different types of GPCRs (such as AT1 or AT2). As previously described, upon binding, activation, and GTP-exchange by the GPCR, the GTP-bound G protein α subunit dissociates from the βγ complex. The Gα subunit binds and activates the enzyme PLC or phospholipase C (the effector protein). PLC cleaves phosphatidylinositol 4,5-bisphosphate (PIP2 or PI(4,5)P2) into principal products, diacylglycerol (DAG) and inositol 1,4,5-trisphosphate (IP3) (Kadamur & Ross, 2013).
The fate of both of these products has substantial effects on the cell. IP3 binds to corresponding IP3-gated Ca2+ release channels on the endoplasmic reticulum (ER) membrane, leading them to open. The Ca2+ stored in the ER is released into the cytosol and can bind a number of protein targets including protein kinase C (PKC).
DAG is another product produced following PLC enzymatic action. DAG is embedded in the inner leaflet of the plasma membrane. DAG activates PKC which proceeds to phosphorylate various targets on Ser and Thr residues. Ca2+-bound PKC is capable of engaging with DAG through its DAG-binding domain (Figure 3.6).
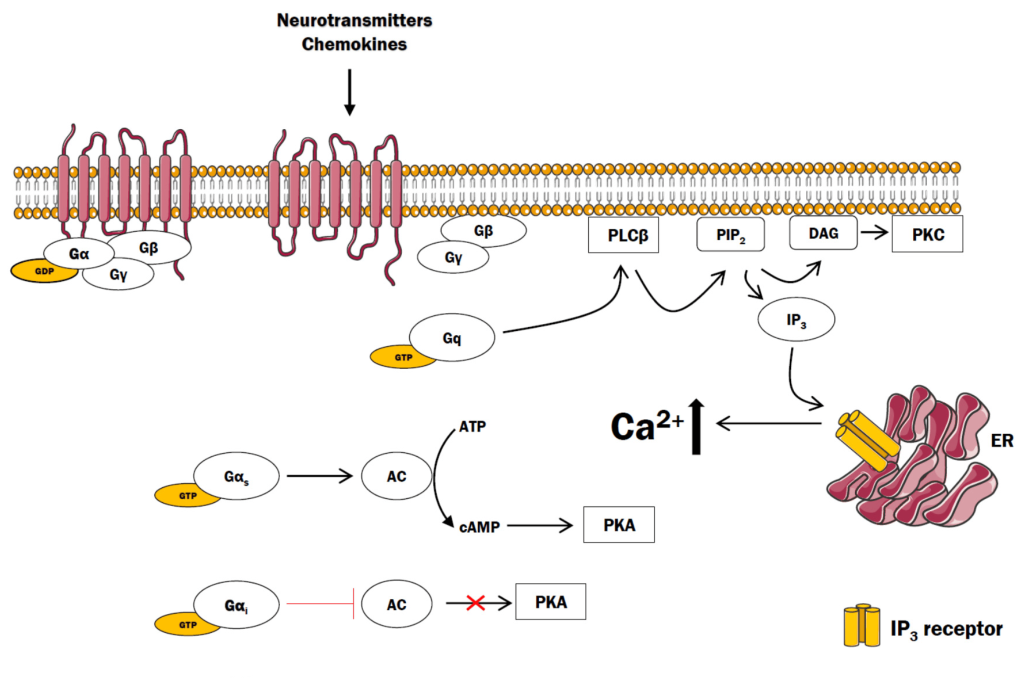
3.4.14 Where is the substrate PIP2 located?
Recall, the structure of the cell membrane is a lipid bilayer is composed of an inner and outer membrane or leaflet. PIP2 is found on the inner membrane leaflet.
3.4.15 PLC has been categorized as the effector in this pathway. What is the secondary messenger?
a. IP3
b. DAG
c. Both a and b
Both IP3 and DAG are examples of secondary messengers, where their cellular concentration increases in response to GPCR signalling pathway activation.
3.5 Biochemical Signalling Pathways are Interconnected
For simplicity, signalling pathways are often introduced as linear pathways that operate both sequentially and in isolation. However, this is rarely the case, and signalling pathways are often interconnected and lead to an intricate upregulation and downregulation of many different proteins (e.g. Figure 3.7). The activity of a single target is often governed by the consensus action of many different effector proteins. There are activators, inhibitors, chaperones, and other proteins that serve as regulators at different points along each biochemical step. This provides a high degree of regulation to precisely control the resulting physiological output from the pathway.
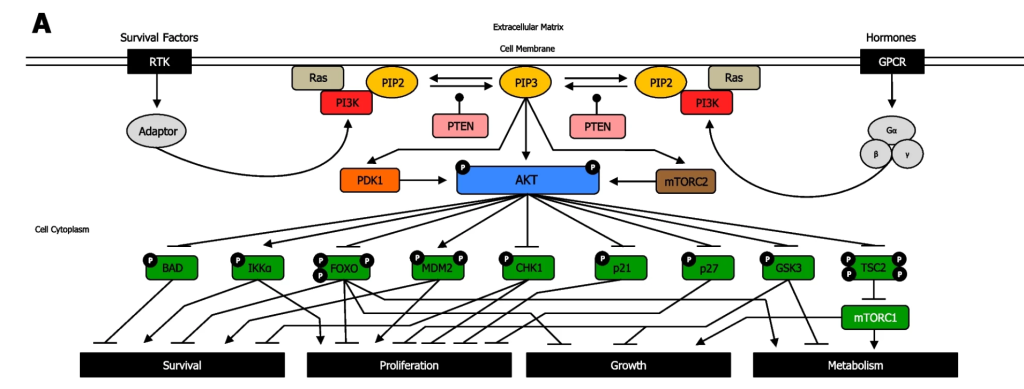
3.5.1 Examine Figure 3.7. What does the uppercase P represent?
The uppercase P symbol indicates that the protein is phosphorylated.
3.5.2 When reviewing the PI3K/Akt pathway in Figure 3.7, notice the blunt arrow from Akt (in the phosphorylated state) to BCL2 associated agonist of cell death (BAD) protein. Which statement best suits the pathway?
a. Akt phosphorylates the protein BAD which results in BAD inhibition
b. Akt inhibits the BAD gene
c. Akt phosphorylates the protein BAD which results in BAD activation
d. Akt activates the BAD gene
A blunt arrowhead indicated that Akt-P inhibits the BAD protein, whereas a normal arrowhead indicates the reaction proceeds as normal. Akt phosphorylates the protein BAD which results in BAD inhibition
3.5.3 What does the arrow in Figure 3.7 from phospho-Akt to IKKα represent?
a. Akt Phosphorylates the protein IKKα inhibiting it
b. Akt inhibits the IKKα gene (CHUK gene)
c. Akt phosphorylates the protein IKKα activating it
d. Akt activates the IKKα gene (CHUK gene)
The arrow indicates that Akt phosphorylates the protein IKKα, thereby activating the protein.
3.5.4 Describe the PI3K/Akt pathway and how it relates to receptors.
Both RTKs and GPCRs are involved in regulating the PI3K/Akt pathway. For both receptors, the cognate ligand can bind and induce a conformational change that will impact and converge on downstream kinases – in particular the PI3K. In the case of GPCRs, it will activate PI3K, whereas in RTKs, a kinase cascade will eventually lead to activation of PI3K.
PI3K phosphorylation results in the formation of PIP3. PIP3 binds to proteins including Akt (PKB) and PDK1. The Ser/Thr kinase Akt, phosphorylates a number of downstream targets resulting in either their activation or inhibition, impacting a plethora of cellular processes. Akt phosphorylates an array of proteins such as Bcl-2-associated death promoter (BAD), forkhead box transcription factors (FOXO), Checkpoint kinase 1 (CHK1), p21, p27, Glycogen synthase kinase 3 (GSK-3) inhibiting them (Figure 3.7). Akt also phosphorylates the protein, Tuberous Sclerosis Complex 2 (TSC2), leading to inhibition, which enables mTOR complex1 to be activated. Conversely, Akt phosphorylation activates IκBα-kinase-α (IKKα) and, Mouse double minute 2 homolog (MDM2).
3.5.5 Using Figure 3.7, how can the effect of kinases be reversed?
The phosphatase PTEN removes phosphate groups from PIP3 to form PIP2.
3.5.6 Following treatment with the inhibitor Bisperoxovanadium, what would be the effect on levels of phosphorylation shown in Figure 3.7:
a. Increase
b. Decrease
c. Observe no impact on phosphorylation levels
Bisperoxovanadium, is a PTEN inhibitor (Schmid et al., 2004) and it would block the phosphatase activity of the protein. It is predicted that phosphorylation levels would increase.
References
Boczek, T., Mackiewicz, J., Sobolczyk, M., Wawrzyniak, J., Lisek, M., Ferenc, B., Guo, F., & Zylinska, L. (2021). The Role of G Protein-Coupled Receptors (GPCRs) and Calcium Signaling in Schizophrenia. Focus on GPCRs Activated by Neurotransmitters and Chemokines. Cells, 10(5). https://doi.org/10.3390/cells10051228
Calebiro, D., Koszegi, Z., Lanoiselée, Y., Miljus, T., & O’Brien, S. (2021). G protein-coupled receptor-G protein interactions: a single-molecule perspective. Physiological Reviews, 101(3), 857–906. https://doi.org/10.1152/physrev.00021.2020
Gaitonde, S. A., & González-Maeso, J. (2017). Contribution of heteromerization to G protein-coupled receptor function. Current Opinion in Pharmacology, 32, 23–31. https://doi.org/10.1016/j.coph.2016.10.006
Glaviano, A., Foo, A. S. C., Lam, H. Y., Yap, K. C. H., Jacot, W., Jones, R. H., Eng, H., Nair, M. G., Makvandi, P., Geoerger, B., Kulke, M. H., Baird, R. D., Prabhu, J. S., Carbone, D., Pecoraro, C., Teh, D. B. L., Sethi, G., Cavalieri, V., Lin, K. H., … Kumar, A. P. (2023). PI3K/AKT/mTOR signaling transduction pathway and targeted therapies in cancer. Molecular Cancer, 22(1), 1–138. https://doi.org/10.1186/s12943-023-01827-6
Hauser, A. S., Attwood, M. M., Rask-Andersen, M., Schiöth, H. B., & Gloriam, D. E. (2017). Trends in GPCR drug discovery: new agents, targets and indications. Nature Reviews. Drug Discovery, 16(12), 829–842. https://doi.org/10.1038/nrd.2017.178
Kadamur, G., & Ross, E. M. (2013). Mammalian phospholipase C. Annual Review of Physiology, 75(1), 127–154. https://doi.org/10.1146/annurev-physiol-030212-183750
Maegley, K. A., Admiraal, S. J., & Herschlag, D. (1996). Ras-Catalyzed Hydrolysis of GTP: A New Perspective from Model Studies. Proceedings of the National Academy of Sciences – PNAS, 93(16), 8160–8166. https://doi.org/10.1073/pnas.93.16.8160
Montminy, M. R., Gonzalez, G. A., & Yamamoto, K. K. (1990). Regulation of camp-inducible genes by creb. Trends in Neurosciences, 13(5), 184–188. https://doi.org/10.1016/0166-2236(90)90045-C
Schmid, A. C., Byrne, R. D., Vilar, R., & Woscholski, R. (2004). Bisperoxovanadium compounds are potent PTEN inhibitors. FEBS Letters, 566(1–3), 35–38. https://doi.org/10.1016/j.febslet.2004.03.102
Seok, S.-H. (2021). Structural Insights into Protein Regulation by Phosphorylation and Substrate Recognition of Protein Kinases/Phosphatases. Life, 11(9). https://doi.org/10.3390/life11090957
Weis, W. I., & Kobilka, B. K. (2018). The Molecular Basis of G Protein–Coupled Receptor Activation. Annual Review of Biochemistry, 87(1), 897–919. https://doi.org/10.1146/annurev-biochem-060614-033910
Welsh, C. L., Conklin, A. E., & Madan, L. K. (2023). Interaction Networks Explain Holoenzyme Allostery in Protein Kinase A. Kinases and Phosphatases, 1(4), 265–287. https://doi.org/10.3390/kinasesphosphatases1040016